Blood-brain barrier
The selective physiological barrier between the fluid spaces of the bloodstream and the central nervous system is referred to as the blood-brain barrier , also blood-brain barrier , or blood-brain barrier .
This particular demarcation of the blood (intravascular) from the extravascular space in the brain and spinal cord is developed in all terrestrial vertebrates (Tetrapoda) and enables the nervous tissue to maintain its own environmental conditions ( homeostasis ). Essentially, this barrier is formed by endothelial cells , which are closely linked here in the capillary blood vessels via tight junctions .
The blood-brain barrier protects the brain from pathogens , toxins and messenger substances circulating in the blood . It is a highly selective filter through which the nutrients required by the brain are supplied and the metabolic products that are created are removed. The supply and disposal is guaranteed by a series of special transport processes .
On the other hand, this protective function of the brain complicates the drug treatment of a large number of neurological diseases , since many active substances cannot cross the blood-brain barrier either. The crossing of the blood-brain barrier is a current research topic in order to be able to treat these diseases. Only very few - extremely rare - diseases are directly related to the blood-brain barrier, while it can itself be affected by a significantly higher number of widespread diseases. A disturbance or damage to the blood-brain barrier caused in this way is a complication to be taken very seriously .
The first experiments that indicated the existence of this barrier were carried out by Paul Ehrlich in 1885. However, he misinterpreted his test results. The final proof of the blood-brain barrier was made in 1967 by electron microscopic examinations.


Functions of the blood-brain barrier
In humans, the brain makes up around 2% of the body mass. However, the proportion of the nutrient requirement is around 20%. In contrast to other organs in the body, the brain has extremely limited reserves of nutrients or oxygen . The nerve cells are also unable to meet their energy requirements anaerobically , i.e. without elemental oxygen. An interruption in the blood supply to the brain leads to unconsciousness after ten seconds and the nerve cells die just a few minutes later. Depending on the activity of a brain area, its energy requirements and reserves can be very different. In order to be able to adapt the supply to the respective needs, these areas regulate their blood supply automatically.
The complex functions of the brain are tied to highly sensitive electrochemical and biochemical processes, which can only run largely without interference in a constant internal environment, the homeostat. For example, fluctuations in the blood pH value must not be passed on to the brain. Fluctuations in the potassium concentration would change the membrane potential of the nerve cells. The neurotransmitters circulating in the blood vessels must not get into the central nervous system, as they would significantly disrupt the flow of information in the synapses present there . In addition, the neurons are not capable of regeneration in the event of damage caused by a fluctuation in the environment. Ultimately, the brain, as a centrally controlling organ, must also be protected from the effects of foreign substances such as xenobiotics and pathogens . The extensive impermeability of the blood-brain barrier to pathogens , antibodies and leukocytes in the blood makes it an immunological barrier .
On the other hand, the high energy requirements of the brain - in comparison to other organs - produce above-average quantities of metabolic degradation products, which have to be removed again via the blood-brain barrier.
In order to guarantee all these functions (supply, disposal and homeostasis), the cerebral blood vessel system of vertebrates shows a number of structural and functional differences compared to the peripheral blood vessel system. This differentiation causes a large separation of the brain from the surrounding extracellular space and is of essential importance for the protection of the sensitive neural tissue as well as for the maintenance of a constant inner milieu.
Changes in the function of the blood-brain barrier cause changes in the state of the central nervous system, which in turn can lead to dysfunction or disease in the CNS. Accordingly, a number of neurological diseases are directly or indirectly related to the blood-brain barrier.
Blood Brain Barrier Anatomy
The essential element of the blood-brain barrier are the endothelial cells with their tight junctions . However, two other cell types, the pericytes and the astrocytes , are of great importance for the function, structure and development of the blood-brain barrier . The cell-cell interactions between endothelial cells, pericytes and astrocytes are closer than in any other cell. These three cell types together form the blood-brain barrier in most vertebrates, the endothelial blood-brain barrier . The following anatomical information relates to the endothelial blood-brain barrier of vertebrates. The glial blood-brain barrier developed in some vertebrates and many invertebrates is listed separately at the end of this chapter.
The endothelium
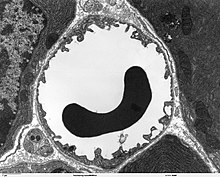
As in the peripheral blood vessels, the capillaries are formed by endothelial cells. The endothelium of peripheral capillaries has openings (fenestrations) of approx. 50 nm diameter and intercellular gaps 0.1 to 1 µm in width for the exchange of water and substances dissolved or suspended in it between the blood and the extracellular fluid of the surrounding tissue. In contrast, there are no fenestrations and no intracellular gaps between the endothelial cells in the brain, which is why one speaks of a continuous endothelium . The (luminal) membrane facing the interior of the capillary differs considerably in terms of the type of membrane proteins from the apical side facing the interstitium .
The number of pinocytotic vesicles that enable endocytosis of dissolved substances is very small in the endothelium of the brain.

In contrast, the number of mitochondria is about 5 to 10 times higher than in the peripheral capillaries. This is an indication of a high energy requirement of the endothelia - among other things for active transport processes - and a high metabolic activity . The blood-brain barrier is not only a physical, but also a metabolic or enzymatic barrier. In the cell membrane of the endothelia there are a number of enzymes in significantly higher numbers than, for example, in the cells of the parenchyma . These include γ-glutamyltransferase , alkaline phosphatase and glucose-6-phosphatase . Metabolizing enzymes such as catechol-O-methyltransferase , monoamine oxidase or cytochrome P450 are also active in the endothelia in relatively high concentrations. In this way, many substances are metabolized before or during their intracellular transport. The endothelial cells are extremely thin at 0.3 to 0.5 µm. In comparison, enterocytes , the epithelial cells of the intestine , are considerably higher at 17 to 30 µm. As with other endothelial cells, the ratio of cholesterol to phospholipids is 0.7. Pure diffusion processes through the cell membrane are therefore just as fast across the blood-brain barrier as with other endothelial cells. The endothelial cells have a large number of aquaporins on their cell membrane for regulating the water balance of the brain . These channels allow water molecules to diffuse freely both towards the brain and towards the blood.
The non-existent fenestrations and the lack of pinocytotic vesicles represent the passive barrier of the blood-brain barrier. This barrier effect can be quantified physically via its electrical resistance . In a healthy adult color rat , the resistance is around 1500 to 2000 Ω cm². In contrast, the value for the capillaries in muscle tissue is around 30 Ω cm².
The active transporter systems of the endothelium, which will be described in more detail later, form the active barrier of the blood-brain barrier.
The tight junctions

A lumen B apical side C basal side a mucus b apical side c basolateral surface d tight junctions f paracellular space g cell membrane |
e protein complex 1 occludin 2 claudin -1 3 E- cadherin 4 ZO-1 5 JAM-1 6 catenins 7 cingulin 8 actin |

The endothelial cells are connected to one another via firm cell-cell connections, the so-called tight junctions . These tight junctions represent dense connections between neighboring cells and make a significant contribution to the barrier function of the blood-brain barrier. This is because they seal the space between the cells and thus practically exclude paracellular transport , the passage of substances along the intercellular space between adjacent endothelial cells past them.
Several transmembrane proteins , such as occludin , various claudins and junctional adhesion molecules (JAM), link the endothelia in the apical area of the lateral cell walls by circumferential protein bands in tight junctions , and thus largely prevent paracellular passage via the intercellular gap between two endothelial cells for larger molecules. The individual protein-protein connections act like fuses connected in parallel. Of the 24 known claudins, the endothelial cells of the blood-brain barrier express only claudin-5 and claudin-12. Claudin-5 is considered to be the most important cell adhesion protein of the blood-brain barrier. Switching off ( gene knockout ) the CLDN5 gene, which codes for claudin-5 , leads to the blood-brain barrier in mice becoming permeable for molecules with a molar mass of up to 800 g · mol −1 . The genetically modified animals died within a few hours of their birth.
The fixation of the endothelia ensures that they are aligned in two very different sides: the luminal side facing the blood and the abluminal side facing the brain.
The basal lamina
The endothelial cells are completely surrounded by a protein layer, the basal lamina. This double layer is about 40 to 50 nm thick and only visible under an electron microscope. It essentially consists of type IV collagen , heparin sulfate - proteoglycans , laminin , fibronectin and other extracellular matrix proteins . The basal lamina borders on the plasma membrane of the terminal feet of the astrocytes.
The pericytes
Pericytes, especially in the older literature after their discoverer Charles Marie Benjamin Rouget (1824–1904) referred to as Rouget cells , are an important component of the blood-brain barrier. Three essential properties are in the foreground: their ability to contract, the regulation of the endothelia and their macrophage activity.
About 20% of the outer endothelial surface of the cerebral capillaries is covered by the relatively small, oval-shaped pericytes and every second to fourth endothelial cell is connected to a pericyte. The pericytes are preferably located at the contact point between two endothelial cells. Pericytes can be found on almost all arterioles, venules and capillaries - not just in the cerebral endothelium. The degree of coverage of the endothelium with pericytes correlates directly with the impermeability of the tight junctions . In fenestrated capillaries, for example in skeletal muscles , the ratio of endothelia to pericytes is 100: 1.
Like the endothelia, the pericytes are also surrounded by a continuous basal lamina.
The cell contact pericyte-endothelium
The pericytes are firmly anchored to the endothelial cells. At least three types of pericyte-endothelial contact form the connection between these two cell types. These are gap junctions (channel-forming protein complexes), focal adhesion ( adhesion plaques ) and peg-and-socket intussusception . The gap junctions connect the two cytoplasms directly and enable the exchange of ions and smaller molecules between the two cell types via channels. The pericytes are mechanically firmly anchored to the endothelia via the focal adhesion. The peg-and-socket invaginations consist of mutually interwoven protuberances of the two interconnected cells. This type of cell contact also obviously contributes to the mechanical anchoring of the pericytes on the endothelia.
Both cell types mutually influence one another with regard to their mitosis and presumably also with regard to their phenotypic expressions .
contraction
Pericytes have a high proportion of the contractile structural protein actin . This allows you to change the capillary diameter via their extensions and thus regulate the blood pressure locally.
Macrocytic Properties
Obviously, only the pericytes on the cerebral capillaries have a special property. There they act as macrophages . There are many lysosomes in the cytoplasm of the pericytes and in vivo they show the ability to take up soluble tracers that are administered intravenously or intraventricularly and are otherwise only taken up by macrophages. The ability of pericytes to phagocytose and to present antigens was demonstrated in tissue cultures .
These macrocytic properties are a "second line of defense " against neurotoxic molecules that have already passed the endothelial layer towards the brain. Pericytes therefore make a significant contribution to the immune system of the central nervous system. On the other hand, through these properties, they are also involved in the development of various diseases, especially autoimmune diseases . An indirect involvement in Alzheimer's disease is also discussed.
Other properties of the cerebral pericytes
The pericytes regulate cell division and the differentiation of the endothelia. In addition, they are precursor cells ( progenitor cells ) formed from pluripotent adult stem cells that can differentiate into osteoblasts , adipocytes , chondrocytes and fibroblast-like cells. Some authors therefore refer to pericytes as pluripotent.
Pericytes synthesize a number of vasoactive agonists and play an important role in the formation of new blood vessels ( angiogenesis ).
The astrocytes
Astrocytes are star-shaped, branched cells from the macroglia family that are significantly larger than the pericytes . They are assigned to the central nervous system and are still capable of dividing after birth. In higher vertebrates they have no direct barrier function, even if they cover about 99% of the capillaries in the brain with their terminal feet. However, they are in direct interaction with the endothelia. Astrocytes induce the function of the blood-brain barrier in the endothelia of the cerebral blood vessels. This has been proven by transplant trials. Cerebral blood vessels that were transplanted into peripheral organs behaved like the “normal” capillaries there and, for example, formed fenestrations. In the reverse experiment, peripheral capillaries that were transplanted into the central nervous system assumed the phenotype there with tight junctions. The influence of astrocytes on the phenotypic expression of the endothelia can also be seen in in-vitro tests. In co-cultures from astrocytes and endothelial cells, the endothelia are more dense than in pure endothelial cultures .
Astrocytes release a number of messenger substances that can modulate the permeability of the endothelium in the range of seconds to minutes. Conversely, the endothelial cells secrete leukemia-inhibiting factor (LIF), a cytokine of the interleukin-6 class, which induces the differentiation of astrocytes. The distance between the astrocyte feet and the endothelial cells and pericytes is only 20 nm.
The main task of the astrocytes, however, is the selective supply of the neurons with nutrients and the regulation of the extracellular ion concentrations. Most of the cholesterol in the brain is produced by astrocytes. Cholesterol cannot cross the blood-brain barrier, which is why it has to be synthesized locally within the brain. About 25% of the body's cholesterol is found in the brain; essentially in the myelin that coats the axons of the neurons.
The terminal feet of the astrocytes form a delicate rosette-like contact with the endothelial cells. This arrangement is important for the mutual influencing and communication of the two cell types. Free diffusion between the endothelium and the parenchyma of the brain is also possible.
Diseases that directly or indirectly affect the astrocytes, such as Alzheimer's disease or astrocytomas , can significantly impair the functioning of the blood-brain barrier due to the close interaction between astrocytes and endothelium.
Areas of the brain without a blood-brain barrier
Not all capillaries in the brain are built up as blood-brain barriers. For example, areas of the brain that release neuroendocrine compounds ( hormones ) into the blood or that have a sensory function - for example for certain peptide hormones - are necessarily without a blood-brain barrier. This is the case with six circumventricular organs . The pineal gland , the eminentia mediana , the neurohypophysis , the area postrema , the organum vasculosum laminae terminalis and the organum subfornicale have fenestrated capillaries. For example, as the neurons of the can area postrema detect for the body of toxic substances and the vomiting center a nausea stimulate. In the organum subcommissurale , the blood-brain barrier is the only one of the circumventricular organs. To protect the surrounding brain tissue, the circumventricular organs are surrounded by tanycytes . These are special ependymal cells with very dense tight junctions.
Blood-brain barrier data
A network of over 100 billion capillary vessels, the total length of which is approximately 600 km for an adult, runs through the entire brain. The average distance between these blood vessels is 40 µm. The different brain regions are supplied to different degrees. The density of capillary vessels is highest in the cerebral cortex ( cortex cerebri ) with 300 to 800 capillary cross-sections per mm² of tissue.
The total area of the blood vessels in the brain is between 12 and 20 m². About 610 ml of blood flow through these vessels per minute, the mean flow rate being 1 mm / s. The mean blood pressure is in the range from 15 to 35 mmHg . The mean transit time ( mean transit time MTT) is 5 seconds. In comparison, it takes about 40 hours in the intestine, whose blood vessels have a surface area of 180 m², and 30 seconds in the liver - with 70 m².
The development of the blood-brain barrier
Up until the end of the 20th century it was assumed that the blood-brain barrier in fetuses and newborns was not yet fully developed (“immature”) or even lacking. The reason for this opinion, which is still widespread today, are methodological inadequacies in earlier physiological experiments. In most cases, protein-binding dyes or proteins themselves were injected into animal fetuses as markers. The first attempts to do this were carried out as early as 1920. In these experiments the markers were detected in the brain or in the CSF of the fetuses, while they were not detectable in the adult animals. However, either volumes were injected, some of which reached the blood volume of the test animals, or the binding capacity of the plasma proteins was exceeded by a factor of two. In both cases, the osmotic pressure is so high that the sensitive embryonic capillary vessels can partially tear. In experiments with reduced marker volumes, no passage of the markers into the brain was found.
If one looks at the body's own marker molecules, such as albumin , α-1-fetoprotein or transferrin , which are found in high concentrations in the plasma even at a very early embryonic stage, these molecules cannot be detected in the extracellular space of the brain at any time. The efflux transporter P-glycoprotein is also already present in the embryonic endothelia. The blood-brain barrier is therefore already present in the prenatal stage. However, this does not rule out changes, especially at the tight junctions, in the course of embryonic development. The tight junctions themselves are present between the endothelial cells at an extremely early stage, but are subject to progressive development.
For small polar molecules such as inulin or sucrose , the permeability of the prenatal and neonatal blood-brain barrier is significantly higher than that of the adult. The same effect can also be observed with ions. The transport of amino acids across the embryonic blood-brain barrier is - obviously due to the great need for further development of the brain - also significantly increased. The same applies to the hormone insulin.
On the other hand, the embryonic brain forms additional morphological barriers that are no longer present in the adult brain. In the embryonic brain, for example, there are so-called strap junctions on the inner ependyma at the interface between liquor and brain tissue .
The blood-brain barrier in the animal kingdom and in the course of evolution

In the course of evolution, the neural tissue of invertebrates and vertebrates became larger, more complex and assumed an increasingly central role in the respective organism. As a result, the coordination of the body functions could be continuously improved. This in turn leads to an advantage in the selection. On the other hand, a brain that is becoming larger and more complex must also be better supplied with nutrients and freed from metabolic products. In the case of more highly developed invertebrates, for example from the order of the decapods or the class of the cephalopods , and all vertebrates, this is ensured by a widely ramified capillary network in the brain. The formation of a protective barrier against xenobiotics, toxins and other substances harmful to the neuronal system is another evolutionary advantage.
Many invertebrates do not have a blood-brain barrier. This means that the endothelia are usually only incompletely lined or with gaps. In invertebrates with a blood-brain barrier, such as insects , crayfish (Crustacea) and cephalopods (Cephalopoda), it is exclusively glial in nature. In these cases one speaks of a glial blood-brain barrier .
The model organism Drosophila , for example, forms a glial blood-brain barrier.
All vertebrates have a blood-brain barrier, and almost all of them form the endothelial blood-brain barrier described in detail in the previous chapters, in which the tight junctions of the endothelia make the essential contribution to the barrier effect. The barrier effect of the blood-brain barrier is only guaranteed by the perivascular astrocytes in the subclass of the slab gill (Elasmobranchii), which includes sharks and rays , as well as the family of sturgeon . It is assumed that, in the course of evolution, the endothelium, if it was dense enough, took over the barrier effect. The glial blood-brain barrier is therefore also regarded as the original form of the blood-brain barrier. In the family of the pike fish (Polypteridae) and the order of the lung fish , the blood-brain barrier is formed by the tight junctions of the endothelia. In contrast, in the real sturgeon it is formed by a complex covering of glial cells without any recognizable tight junctions.
Today found in some vertebrates elements of glial barrier, such as in the glial limiting membrane of the avascular (avascular) spinal cord of the lamprey and in mammals in certain ependymialen structures such as the Tanyzyten, the latter especially in the circumventricular organs, the choroid plexus and the pigment epithelium the retina (RPE). Astrocytes are in principle able to form tight junctions with each other, even in mammals. This can be demonstrated, among other things, in the olfactory envelope cells (OEC, English olfactory ensheathing cells ), which enclose growing axons.
From the structural differences in the phenotype of the glial blood-brain barrier in invertebrates, it can also be deduced that such barriers arose several times and independently of one another in the course of evolution . The endothelial barriers offer a significant advantage in selection - presumably due to the stricter separation of the function of endothelium and astrocytes. The main selection pressure probably came from the need for homeostasis. It is believed that the endothelial blood-brain barrier developed at least six times in the course of evolution and that all vertebrates 400 to 500 million years ago were provided with a glial blood-brain barrier.
Blood-liquor barrier
In addition to the blood-brain barrier, the blood-liquor barrier is the second border between the blood circulation and the central nervous system . This blood-liquor barrier is formed by epithelial cells and tight junctions of the choroid plexus . The blood-liquor barrier also ensures the homeostasis of the central nervous system. Only low molecular weight substances can pass through them as ultrafiltrates from the intravascular space of the blood vessels into the liquor space or those that are actively absorbed and secreted by the ependymal cells of a choroid plexus. However, the contribution to the exchange of substances is small and can by no means meet the needs of the brain. The exchange area of the plexus epithelia is about 5000 times smaller than that of the intracerebral capillary network that forms the blood-brain barrier.
In addition to these two barriers that are so important for the central nervous system, other similar, highly selective barriers can be found in the human body that control the exchange of substances via the blood. These include the blood-placenta barrier , the blood-testis barrier , the blood-urine barrier , the blood-eye barrier , the blood-thymus barrier and the blood-air barrier .
Transport processes of the blood-brain barrier
Despite its function as a protective barrier, the blood-brain barrier must also ensure the transport of nutrients to the brain and the removal of metabolic products from the brain. Water-soluble nutrients and peptides cross the blood-brain barrier mainly through specific transporters or special channels in the cell membrane. Most other soluble compounds pass, if at all, this barrier by diffusion.
Paracellular transport
In peripheral capillaries, substances are transported to organs and muscles mainly through fenestrations and intracellular fissures. In a healthy and intact cerebral endothelium, the individual endothelial cells are tightly connected to one another via the tight junctions. The capillary vessels of the brain therefore mainly allow only a transmembrane transport of substances, which can also be better regulated by the cells than the paracellular transport. Water, glycerine, and urea are examples of small polar compounds that can diffuse through the tight junctions of the blood-brain barrier.
Free diffusion

The simplest form of transport through the blood-brain barrier is free diffusion . This exchange, also known as passive transport, can in principle take place both through the cell membrane of the endothelia and through the tight junctions . As with every diffusion, an equalization of the concentration or the equalization of an electrochemical gradient is sought. With free diffusion, no energy is required from the cell for membrane transport. The material flow is proportional to the concentration and cannot be regulated by the cell.
The lipophilic (“fat-friendly”) properties of the cell membrane and their close connection via tight junctions reduce the number of substances that can cross the blood-brain barrier by diffusion, however, considerably. The permeability of the blood-brain barrier for a particular molecule is directly related to its lipophilicity. With regard to the molar mass , it is inversely proportional. That is, the more lipophilic and smaller a compound is, the easier it can diffuse through the endothelium. A maximum size of 400 to 500 g · mol −1 with an intact blood-brain barrier is given as the limit value for the molar mass of a molecule . Molecules above this limit cannot diffuse across the blood-brain barrier. However, the blood-brain barrier should not be understood as a discrete barrier that completely retains a certain size of molecules and allows a smaller one to completely diffuse into the brain. The diffusion processes at the blood-brain barrier are dynamic equilibria. For a molecule with a cross-sectional area of 0.52 nm², which corresponds to a molar mass of about 200 g · mol −1 , the blood-brain barrier is 100 times more permeable than for a molecule with an area of 1.05 nm² (= 450 g mol −1 ).

In 1971 the Göttingen biophysicist Hermann Träuble developed a theory about the transport of small molecules through the cell membrane. According to this, small cavities located between the fatty acid chains of the lipid bilayer are responsible for the diffuse transport. The cavities are created by transitions from the anti - to gauche - conformation ( trans-gauche-trans Kinks ) in the fatty acid residues of the phospholipids of the cell membrane. There are only very low energy thresholds between these conformational transitions. The Kinks (engl. Kink , bend ') are mobile and migrate with the molecule to be transported through the membrane. Träuble's theory was confirmed by NMR spectroscopy by Anna and Joachim Seelig in 1974 .
With regard to lipophilicity, the partition coefficient in octanol / water is an important indicator of the ability of a substance to diffuse through the blood-brain barrier. The partition coefficient is usually in logarithmic form as log P stated. A log P value of a substance of, for example, 3.8 means that this substance is distributed in octanol (lipophilic) in a concentration that is 10 3.8 times higher than in water (hydrophilic). If the log P value is 0, the substance is distributed equally in both phases; if it is negative, the substance is hydrophilic. In principle, lipophilic substances can most easily pass through the cells' plasma membrane, which is made up of fatty acids. If the log P value is above 3, this effect usually decreases again, since such molecules have a high binding affinity for plasma proteins .
The prediction of the ability of a substance to penetrate the brain is possible using various models and simulations ex vivo or in silico . Reliable in-vitro studies can be carried out on isolated endothelial cell cultures.
Lipophilicity and small molecule size are no guarantees of possible diffusion to the brain. For example, over 98% of all low molecular weight pharmaceuticals ( small molecules ) are unable to cross the blood-brain barrier. High molecular substances such as monoclonal antibodies , recombinant proteins , antisense RNA or aptamers are retained by the blood-brain barrier per se.
Channel-mediated permeability
Small polar molecules, such as water, can only diffuse through the endothelium to a very limited extent via the hydrophobic kinks . Nevertheless, the blood-brain barrier has a very high permeability for water. This was demonstrated by William Henry Oldendorf (1925–1992) in 1970 in experiments with tritium- labeled water.
For the diffusion of water there are special hydrophilic channel proteins in the cell membrane, the aquaporins . While non-cerebral endothelia very often express aquaporin-1 (AQP1), this gene is inactivated at the blood-brain barrier. The presence of the astrocytes inhibits AQP1 expression. The specialized cerebral endothelia mainly express aquaporin-4 (AQP4) and aquaporin-9 (AQP9).
The water balance of the brain is regulated via the aquaporins. They allow a high capacity for the diffusion of water in both directions, following the osmotic gradient. For glycerol, urea and monocarboxylates forming Aquaglyceroporin own channels in the plasma membrane. At the blood-brain barrier, this is essentially aquaporin-9, which is also responsible for water transport.
Channels are much faster than transporters for molecule transport. The ion channels can be activated or deactivated ( gating ) by voltage pulses or interacting hormones and other influencing factors .
Facilitated diffusion
A special form of diffusion through the cell membrane of the endothelial cells is the facilitated diffusion (engl. Facilitated diffusion ). Vital nutrients such as glucose and many amino acids are too polar and too large to be made available to the brain in sufficient quantities via the blood-brain barrier on the previously described transport routes. There is a special transport system for these molecules in the cell membrane: the so-called carrier-mediated transport . For example, D- glucose is transported into the brain via the GLUT-1 transporter. The density of GLUT-1 transporters is four times higher on the abluminal side of the endothelia than on the luminal side, i.e. the side facing the blood. The transport is only made possible by a concentration gradient and does not require any energy itself.
In addition to the D- glucose required by the brain in relatively large quantities, there are a number of other special transporters in addition to GLUT-1. Many of these transporters are from the Solute Carrier (SLC) family, including MCT-1 and MCT-2, which transport a number of short-chain monocarboxylic acids - including lactate , pyruvate , mevalonate , various butyrates and acetate . SLC7 transports cationic amino acids ( arginine , lysine and ornithine ). To date, 307 SLC genes are known from the mouse model organism . Of these, over 200 are expressed in the choroid plexus and other brain areas. However, so far only 36 have been detected at the blood-brain barrier of the same species . About 70% of the 36 have gene products that are involved in the transport of nutrients, vitamins, hormones and trace elements. The thyroid transporters SLC16a2 and SLCO1c1, the sulfate transporter SLC13a4, the L - ascorbic acid transporter SLC23a2, the amino acid transporter SLC 38a3 and the folate transporter SLC19a1 are particularly strongly expressed at the blood-brain barrier . Ascorbic acid (vitamin C) is supplied to the brain in oxidized form via the glucose transporter.
The transporters can work as a uniporter (only in one direction), as a symporter in two directions and as an antiporter in the opposite direction.
Active transportation
In the passive types of transport through the endothelium described above, the molecules get to the brain or away from the brain without any additional need for energy. They follow the respective concentration gradient. With active transporters, so-called "pumps", transport is also possible against a concentration gradient. However, this directly or indirectly requires energy in the form of adenosine triphosphate. If the active transport takes place from the blood to the brain, one speaks of influx ("inflow"). In the opposite direction, one speaks of efflux ("drainage").
At the blood-brain barrier there are active influx transporters for leu-enkephalin , arginine-vasopressin (AVP) and [D-penicillamin2, D-penicillamin5] -enkephalin (DPDPE).
P-glycoprotein - the gene product of the MDR1 gene - was the first efflux transporter to be identified at the blood-brain barrier. The multidrug resistance-related proteins , for example MRP1, which also belong to the class of ABC transporters , were later added . The Breast Cancer Resistance Protein ( Breast Cancer Resistance proteins , BCRP) is located together with the P-glycoprotein essentially on the blood directed towards the luminal side of the endothelium.
Some of the efflux transporters - like some of the influx transporters - work stereoselectively . This means that they only transport one enantiomer from the brain into the blood vessel system. D - aspartic acid is a precursor of N -methyl- D -aspartate (NMDA) in the brain and influences the secretion of various hormones, such as luteinizing hormone , testosterone or oxytocin . The L contrast aspartic belongs together with L - glutamic acid to the excitatory amino acids. The efflux transporter ASCT2 (alanine-serine-cysteine transporter) of the blood-brain barrier only transports the L -enantiomer of aspartic acid, the accumulation of which would have neurotoxic effects. In contrast , the D enantiomer required for the construction of NMDA is not transported by ASCT2. The EAAT transporters ( excitatory amino acid transporters ) SLC1A3, SLC1A2 and SLC1A6, on the other hand, transport both isoforms of aspartic acid.
In epileptic tissue, P-glycoprotein is overexpressed in the endothelia and astrocytes of the blood-brain barrier and in the neurons.
Furthermore, the organic anion transporters (OAT and OATP) and organic cation transporters (OCT) are located in the cell membrane of the endothelia. The efflux transporter in particular can actively transport a large number of different substrates from the endothelium into the capillaries.
For a number of transport processes on the endothelium, it is still unclear whether they are active (ATP-consuming) or carrier-mediated processes.
Vesicular transport
Receptor-mediated transcytosis
There is receptor-mediated transcytosis for the transport of selected large molecules . Special receptors that protrude through the cell membrane into the lumen are responsible for recognizing the substances to be absorbed. Thus, for example, passes the 75.2 kDa heavy and consisting of 679 amino acids, transferrin from the blood into the extracellular fluid of the brain. The receptors in the lumen are internalized after the transferrin has been attached, that is, they are introduced into the cell interior. It is then transported to the other side of the cell (abluminal side) and discharged via coated pits . The same mechanism enables the transport of low-density lipoprotein to the brain via the LDL receptor in order to be able to produce cholesterol there . Also, insulin and other peptide hormones and cytokines go on this way to the brain.
Adsorption-mediated transcytosis
In adsorptive-mediated transcytosis ( AMT), electrostatic interactions between the cell surface negatively charged by glycoproteins and positively charged molecules ( cations ) cause transport through the cytoplasm of the endothelia. This form of transport is also known as cationic transport . Peptides and proteins, for example, whose isoelectric point is basic, have a positive charge . Cationic transcytosis through the endothelium of the blood-brain barrier enables a higher degree of substance transport than receptor-mediated transcytosis.
The most important transporters at the blood-brain barrier
Transporter | Designation (english) | gene | Chromosome, gene locus | family | source |
Facilitated diffusion | |||||
GLUT1 | glucose transporter 1 | SLC2A1 | 1 p35-p31.3 | solute carrier | |
LAT1 | large neutral amino acid transporter 1 | SLC7A5 | 16 q24.3 | solute carrier | |
CAT1 | cationic amino acid transporter 1 | SLC7A1 | 13 q12.3 | solute carrier | |
MCT1 | monocarboxylic acid transporter 1 | SLC16A1 | 1 p13.2-p12 | solute carrier | |
CNT2 | concentrative nucleoside transporter 2 | SLC28A2 | 15 q15 | solute carrier | |
CHT1 | choline transporter 1 | SLC5A7 | 2 q12 | solute carrier | |
NBTX | nucleobase transporter | ? | ? | ||
Active efflux | |||||
MDR1 | P-glycoprotein | ABCB1 | 7 q21.1 | ATP-binding cassette | |
MRP1 | multidrug resistance protein 1 | ABCC1 | 16 p13.1 | ATP-binding cassette | |
MRP3 | multidrug resistance protein 3 | ABCC3 | 17 q22 | ATP-binding cassette | |
MRP4 | multidrug resistance protein 4 | ABCC4 | 13 q32 | ATP-binding cassette | |
MRP5 | multidrug resistance protein 5 | ABCC5 | 3 q27 | ATP-binding cassette | |
BCRP | breast cancer resistance protein | ABCG2 | 4 q22 | ATP-binding cassette | |
OAT3 | organic anion transporter 3 | SLC22A8 | 11 | solute carrier | |
OATP-A | organic anion transporter polypeptide A | SLC21A3 | 12 p12 | solute carrier | |
OATP3A1 | organic anion transporter polypeptides 3 | SLCO1A2 | 15 q26 | solute carrier | |
EAAT1 | excitatory amino acid transporter 5 | SLC1A3 | 5 p13 | solute carrier | |
TAUT | taurine transporter | SLC6A6 | 3 p25-q24 | solute carrier | |
Receptor-mediated transport | |||||
INSR | Insulin receptor | INSR | 19 p13.2 | ||
TFR1 | transferrin receptor | TFRC | 3 q29 | ||
IGF1R | insuline-like growth factor 1 receptor | IGF1R | 15 q25-q26 | ||
LEPR | leptin receptor | LEPR | 1 p31 | ||
FCGRT | Fc fragment of IgC, receptor transporter | FCGRT | 19 q13.3 | ||
SCARB1 | scavenger receptor class B 1 | SCARB1 | 12 q24.31 |
The interaction of the diverse members of the Solute Carrier family and the ABC transporter is an extremely effective protective mechanism of the blood- brain barrier to prevent xenobiotics from entering the brain.
Measurement and display of the permeability of the blood-brain barrier
In the development of new pharmaceuticals, the extent to which a substance can cross the blood-brain barrier is an important pharmacological parameter ( brain uptake ). This applies both to neuropharmaceuticals , which are supposed to have their effect mainly in the central nervous system, and to pharmaceuticals, which are only supposed to have an effect in the periphery. A number of different methods have been developed in the past to investigate whether, to what extent and with which mechanism a substance can cross the blood-brain barrier. The classic methods work with model organisms in vivo . Newer approaches use cell cultures ( in vitro ) and a very new method works via computer simulations ( in silico ). Due to the largely identical structure of the blood-brain barrier in mammals, the in vivo results can be easily transferred to humans.
Physical basics
A simplified model based on a single capillary was established by Renkin (1959) and Crone (1965) for measurements of the permeability of the blood-brain barrier. Despite the simplifications, this model is the best approximation of the real conditions. The so-called permeability surface ( permeability surface area product ) PS is a measure of the permeability of the capillary bed. The permeability surface product, also known as the Crone-Renkin constant , is the product of the permeability coefficient of a certain substance and the available transfer area. The unit of measurement is ml · min −1 · g −1 (g wet tissue) and corresponds to that of perfusion (blood flow) Q. The one-sided ( unidirectional ) extraction fraction E, that is the proportion of a substance that passes through the brain is transported, then results from:
.
If the perfusion (Q) is greater than the permeability surface product (PS), the transfer of substances is perfusion-limited. If the situation is reversed, the mass transfer is limited by the diffusion.
In principle, the value of E for all substances is always less than 1, since the influx cannot be greater than the supply of substance. At values less than 0.2 it is usually said that permeation is the limiting factor for the transport of substances to the brain. In the range from 0.2 to 0.8 the permeation is moderate.
In-silico procedure
The simulation methods are mainly used in the very early phase of drug development, i.e. drug design . The calculation models are currently limited to passive transport by diffusion and some molecular descriptors , such as lipophilicity, charge, molar mass and number of hydrogen bonds .
In vitro method
The simplest in vitro method is to use isolated, still living capillaries. This makes it possible to investigate the transport mechanisms at the cellular level. In some cases, capillaries of human origin from autopsies are also used. The capillaries are metabolically active even after isolation , even if the ATP supply in the cells is largely depleted. In this method, however, both the luminal and the abluminal side of the endothelia are exposed to the active substance to be examined. It is therefore not possible to differentiate between the two sides with regard to the intake of active substances. The spatial distribution of incubated capillaries can be analyzed using confocal fluorescence microscopy . For example, the uptake of fluorescence- labeled monoclonal antibodies at the transferrin receptor can be examined. The method can be refined so that semi-quantitative assays for the efflux of active ingredients on the luminal side of the endothelia are also possible.
With immortalized endothelial cell lines, quantitative statements can be made about the permeability of a substance. There are now a variety of different cell lines from different species . These cell lines are used for both basic research and drug development. As in the capillary vessels, the endothelial cells are grown in single layers ( monolayers ). The quality of these layers can be assessed, for example, via the electrical resistance of the endothelial layer. The transendothelial electrical resistance ( TEER ) should be as high as possible. In the living organism this value is above 2000 cm² and is essentially determined by the quality of the tight junctions. In vitro values are usually an order of magnitude lower. These values improve significantly when co-cultures of endothelia and astrocytes are used. The astrocytes have a positive effect on the gene expression of the endothelia to form tight junctions. In this way, values of up to 800 cm² can be achieved. Similarly high values can also be achieved without astrocytes if the cell culture contains cortisol .
In vivo method

The first method used by Paul Ehrlich to demonstrate the (im) permeability of the blood-brain barrier was the injection of a dye and the subsequent dissection of the test animal. Dyes that are able to cross the blood-brain barrier permanently stain the interstitium of the brain. The method is limited to dyes and only allows qualitative considerations. Dyes are still used today in studies aimed at opening the blood-brain barrier in a targeted manner. For the same reason, the method of fluorescent labeling, which is otherwise very often used in microbiology, is completely unsuitable for investigating the transport mechanisms of small molecules at the blood-brain barrier. Fluorescent labeling can only be used for very large molecules, such as polypeptides, in which the molecule of the fluorescent dye is relatively small in relation to the peptide.
A number of different in vivo methods have been developed in the past, some of which are still used in practice today. In general, the in vivo methods are the reference methods. The intravenous or intra-arterial application of an active ingredient under real physiological conditions in a model organism and subsequent analysis of the brain tissue cannot currently be replaced by any in-vitro methods or even by simulation calculations. These are the most sensitive methods in which the uptake of a substance in the brain can be determined over long periods of time and several passages through the blood-brain barrier.
Brain uptake index
The first method for quantifying the uptake of nutrients and active substances across the blood-brain barrier was developed in 1970 by William H. Oldendorf. A radioactively marked substance is injected together with a likewise radioactively marked reference sample directly and as quickly as possible into the external carotid artery of a test animal (usually a rat). About five seconds after the injection, the animal is decapitated , the brain is isolated and the concentration of the labeled substances it contains is determined from the radioactivity. The so-called brain uptake index (BUI) is calculated from the ratio of the dose of the injected substances and the dose measured in the isolated brain for test substance and reference sample .
Because of the very short time in which the applied substances are administered and can permeate, the method is only suitable for substances that quickly pass the blood-brain barrier. In contrast, hydrophilic compounds, such as many peptides, are only absorbed slowly. The method is unsuitable for such substances.
The compounds to be investigated are mostly tritium or 14 C labeled. Tritated water or 14 C-butanol , for example, serve as reference substances . The volume injected into the test animal is kept as small as possible. Volumes smaller than 10 µl are necessary in order to avoid flow and distribution artifacts , as these influence the measured BUI. In contrast, the BUI's dependence on the concentration of the injected substance is rather low.
The brain uptake index of various substances
connection | BUI [%] | log P | M [g / mol] |
Cytosine | 1.2 | −1.72 | 243.2 |
urea | 1.4 | −1.52 | 60.1 |
Mannitol | 2 | −2.11 | 182.2 |
Thiourea | 5 | −0.96 | 76.12 |
Ethylene glycol | 18th | −1.3 | 62.1 |
Acetamide | 23 | −1.1 | 59.1 |
Methanol | 103 | −0.52 | 32 |
Propylene glycol | 27 | −0.92 | 76.1 |
Ethanol | 107 | −0.18 | 46.1 |
Butanol | 117 | 0.93 | 74.1 |
Benzyl alcohol | 94 | 1.1 | 108.1 |
phenobarbital | 56 | 1.42 | 232.2 |
Phenazone | 83 | 0.38 | 188.2 |
caffeine | 103 | 0.02 | 194.2 |
Phenytoin | 71 | 2.4 | 252.3 |
Estradiol | 94 | 2.61 | 272.4 |
testosterone | 85 | 3.28 | 288.4 |
heroin | 87 | 1.14 | 369.4 |
Morphine | 2.6 | −0.2 | 285.3 |
nicotine | 120 | 0.7 | 162.2 |
according to:, data, unless otherwise indicated, from:
Brain Efflux Index
In this procedure, developed in 1995, the efflux of a substance from the brain across the blood-brain barrier into the blood is determined.
As with the brain uptake index, the radioactively marked test substance is microinjected directly into the brain of the test animal together with a reference compound that is also radioactively marked. However, a non-permeable tracer - mostly inulin - is used for the reference compound. Here, too, the test animal is decapitated after a certain time and the brain is isolated. The radioactivity is then measured on the same side of the brain ( ipsilateral ). The brain efflux index (BEI) is then calculated from the proportion of the test substance that crosses the blood-brain barrier and circulates in the blood and the proportion remaining in the brain:
- .
Brain perfusion
In the perfusion technique, the radioactively labeled substance to be examined is perfused into the external carotid artery of the test animal. The animal is then killed and the brain removed. The brain is homogenized and the radioactivity in it is measured. The advantage of this method is that a possible breakdown of the test substance in the blood, for example by the enzymes it contains, is largely prevented. Guinea pigs are particularly suitable as test animals because the carotid artery does not branch between the neck and the brain, as is the case, for example, with the rat. This makes it possible to increase the perfusion time up to 30 minutes, while in the rat only 20 seconds are possible. The operational effort of this method is extremely high. Therefore, it is essentially only used for substances that do not have sufficient stability in the plasma or only have a very low perfusion capacity to the brain.
An improved variant, the capillary depletion method (Engl. Capillary depletion method ). The capillaries are separated from the brain tissue by centrifugation . This allows a distinction to be made between transcytosis or diffusion, i.e. actual permeation into the brain, and possible endocytosis in the endothelium.
Indicator diffusion technique
In the indicator diffusion technique , the active ingredient to be examined is applied to the external carotid artery together with a reference substance that has no permeation capacity . Both substances do not have to be radioactively labeled. From the backflow of blood, for example in the internal jugular vein , the concentration of both substances can be determined by taking blood and analyzing the plasma. The permeation of the substance into the brain can be calculated from the decrease in the test substance in the blood reflux.
In the brain, the indicator diffusion technique is only suitable for substances that have a high permeation capacity for this organ.
Quantitative autoradiography
see main article: Autoradiography

Quantitative autoradiography (QAR) was developed in the 1970s. The substance , which is usually radioactively labeled with 14 C, is administered intravenously. At a certain point in time, the organs are isolated and the dried frozen sections are placed on an X-ray film or a high-resolution scintigraphic detector plate. The degree of blackening or the detected radiation dose can be used to measure the absorption of the substance in the brain, but also in other organs, by means of comparative experiments with substances with very low permeability - for example with 125 I -labelled albumin . If the local perfusion is determined in a separate measurement, the permeability surface product can be calculated.
Intracerebral microdialysis
In intracerebral microdialysis , a semi-permeable membrane is implanted in the brain tissue. Substances can be perfused via a microdialysis catheter located inside the membrane . Samples of the perfusate can then be taken and analyzed using the same catheter.
A variety of examinations and experiments at the blood-brain barrier can be carried out in the test animal. An example of this is the opening of the blood-brain barrier using hypertonic solutions, as described below. The method is suitable for continuous fluid withdrawal. In this way, concentration-time profiles can be created for intravenously or orally administered substances.
A wide variety of experiments to research transport mechanisms at the blood-brain barrier can be carried out with intracerebral microdialysis.
In human medicine, intracerebral microdialysis is used for neurochemical monitoring of strokes .
Imaging procedures
With the help of imaging methods , a non-invasive display and measurement of the permeability of the blood-brain barrier with various substances is possible. Some of these methods are used in clinical practice in human medicine. The moreover be used positron emission tomography (PET), the magnetic resonance spectroscopy (MRS) and magnetic resonance imaging (MRI).
- PET

With positron emission tomography, essentially the efflux processes of potential active substances with P-glycoprotein are shown to investigate the blood-brain barrier. Understanding the function and influence of P-glycoprotein at the blood-brain barrier is of great importance for the development of neuropharmaceuticals. These purely scientific investigations are carried out with substances that are marked with a positron emitter (beta plus decay (β +)). The isotope 11 C is mainly used for this. The short-lived isotope 18 F is preferred for fluorine-containing compounds . Because of the very short half-life of 20.39 for 11 C or 110 minutes at 18 F, such experiments can only be carried out at research facilities near which a cyclotron is located. Examples of appropriately marked and investigated compounds are verapamil , carazolol , loperamide and carvedilol . Verapamil is of particular pharmacological interest because it is able to inhibit P-glycoprotein .
PET is one of the few methods that enables a direct in vivo comparison between the model organisms used in the preclinical phase and humans with regard to the interaction between active ingredient and P-glycoprotein.
- Magnetic resonance imaging
Magnetic resonance tomography (MRT) is too insensitive as an imaging method to show the passage of active substances into the brain. The situation is completely different with a damaged blood-brain barrier. In these cases, contrast- enhanced MRI plays an important role as a diagnostic method for various neurodegenerative diseases and cancers in the brain. This is described in more detail in the chapter on diseases indirectly associated with the blood-brain barrier .
- Magnetic resonance spectroscopy

The magnetic resonance spectroscopy (MRS) is a on the nuclear magnetic resonance -based method in a magnetic resonance imaging scanner to carry out a nuclear spin resonance spectroscopy allows. Certain substances or their metabolic products can be spectroscopically detected and quantified in the brain. Compared to magnetic resonance tomography based on the proton spin of water, other atomic nuclei are of interest here. These are in particular 19 F, 13 C, 31 P and non-aqueous protons. Compared to the protons of the water, which are present in much larger quantities, these nuclei, which are usually only present in traces, provide correspondingly weak signals. The spatial resolution corresponds to a volume element of approximately 1 cm³. MRS and MRT can be easily combined with one another. The MRT provides the anatomical structure and the MRS the corresponding spatially resolved analysis of the anatomy. With the MRS, for example, the pharmacokinetics of active ingredients containing fluorine , such as the neuroleptics trifluoperazine and fluphenazine , can be observed and measured in the human brain. With magnetic resonance spectroscopy, it is possible to quantitatively differentiate between the active substance and its metabolites. It is also possible to differentiate between free and bound active substance.
Disadvantages of the MRS are the long measurement times required due to the low sensitivity of the method and the low spatial resolution. The latter is particularly problematic in experiments with small animals.
Concepts for overcoming the blood-brain barrier
see main article: Concepts for Overcoming the Blood-Brain Barrier
As was shown in the chapter on transport processes , only a few substances are able to cross the blood-brain barrier, which is why many potential neuropharmaceuticals ultimately fail at the blood-brain barrier. 98% of these substances cannot cross the blood-brain barrier.
For decades, intensive research has therefore been carried out on methods that are intended to enable the transport of active substances into the brain by bypassing or - ideally more selectively - opening the blood-brain barrier. A number of strategies to overcome the blood-brain barrier have been developed or are still in the development stage.
Disorders of the blood-brain barrier
Main article: Disruption of the blood-brain barrier
Disorders of the blood-brain barrier can be caused by a number of different diseases. The blood-brain barrier itself can also be the starting point for some very rare neurological diseases that are genetic .
The disruption of the protective effect of the blood-brain barrier is a complication of many neurodegenerative diseases and brain injuries. Some peripheral diseases, such as diabetes mellitus or inflammation , have a detrimental effect on the functioning of the blood-brain barrier.
Other diseases, on the other hand, disrupt the function of the endothelia from “inside”, that is, the integrity of the blood-brain barrier is impaired by influences that come out of the extracellular matrix. An example of this is glioblastoma .
In contrast, a number of diseases in the brain manifest themselves in that certain pathogens can cross the blood-brain barrier. These include, for example, the HI virus, the human T-lymphotropic virus 1 , the West Nile virus and bacteria such as Neisseria meningitidis or Vibrio cholerae .
In the case of multiple sclerosis, the "pathogens" are cells of the body's own immune system that cross the blood-brain barrier. Likewise, in some non-cerebral tumors, metastatic cells cross the blood-brain barrier and can lead to metastases in the brain.
Exogenous effects on the blood-brain barrier
alcohol
Alcohol abuse is a major risk factor for neuroethological diseases, inflammatory diseases and susceptibility to bacterial infections. In addition, chronic alcohol consumption damages the blood-brain barrier, which is seen as a major influencing factor for the development of some neurodegenerative diseases. The damage to the blood-brain barrier has been proven by neuropathological studies of alcohol addicts as well as animal experiments.
In animal experiments, it was found that the enzyme myosin light chain kinase (MLCK) activated by alcohol consumption leads to the phosphorylation of several tight junction or cytoskeletal proteins in the endothelia , which affects the integrity of the blood-brain barrier is pulled. In addition, the oxidative stress caused by alcohol leads to further damage to the blood-brain barrier.
It is not the alcohol itself, but its metabolites that activate the MLCK enzyme in the endothelia. The dysfunction of the blood-brain barrier facilitates the migration of leukocytes into the brain, thereby promoting neuroinflammatory diseases.
nicotine
Chronic nicotine abuse not only increases the risk of lung cancer, but also increases the risk of cardiovascular disease . There is, in turn, a direct relationship between cardiovascular risk factors and an increased risk of dementia . In several meta-studies it was found that smokers have a significantly higher risk of dementia from Alzheimer's disease than non-smokers. The risk of vascular dementia and mild cognitive impairment is not or only slightly increased. The chronic administration of nicotine changes both the function and the structure of the blood-brain barrier of the test animals in animal experiments. The model substance sucrose can pass the endothelia significantly more easily, which is essentially due to a changed distribution of the tight junction protein ZO-1 and a reduced activity of claudin-3. Furthermore, after chronic administration of nicotine, increased formation of microvilli , regulated Na + K + 2Cl - symporters and regulated sodium-potassium pumps were observed in the endothelium of the blood-brain barrier .
In epidemiological studies, smokers were found to have a significantly higher risk of developing bacterial meningitis than non-smokers. Nicotine alters the actin filaments of the endothelial cytoskeleton , which apparently facilitates the passage of pathogens such as E. coli to the brain.
For some diffusion-restricted compounds such as the nicotine antagonist methyllycaconitine (MLA), which binds to the nicotine acetylcholine receptor (nAChrs), the blood-brain passage is worsened with chronic nicotine consumption.
A vaccine based on a nicotine-specific immunoglobulin G is under development. The aim of this vaccine is to stimulate the formation of antibodies that specifically bind nicotine and thus prevent passage through the BBB.
Electromagnetic waves (cellular radio)
The negative health effects of electromagnetic radiation in the megahertz to gigahertz range with high energy density have been proven. In contrast, the effects of the same with a lower energy density, as is mainly used in mobile and data radio, are controversially discussed. The effects on the blood-brain barrier are also an issue.
When the energy density of electromagnetic radiation is high, significant warming is observed in the affected body tissue. In the skull, this warming can influence the blood-brain barrier and make it more permeable. Such effects are also demonstrated by the action of heat sources on peripheral parts of the body. With the services used in mobile communications, the brain can be heated by a maximum of 0.1 K (15-minute mobile communications call with maximum transmission power). A warm bath or physical exertion can heat the brain more without harm. In scientific studies since the beginning of the 1990s, in particular from the working group of the Swedish neurosurgeon Leif G. Salford at the University of Lund , results have been obtained that open the blood-brain barrier in the non-thermal range after exposure to GSM . Frequencies, describe. In-vitro experiments on cell cultures later came to similar results.
Other working groups cannot confirm Salford's results. Other working groups also question the methodology used.
Human diagnostics
Contrast enhanced magnetic resonance imaging
Shortly after the development of gadopentetate dimeglumine (Gd-DTPA), the first contrast agent for magnetic resonance tomography, in 1984, the potential of contrast-enhanced magnetic resonance imaging for the diagnosis of local disorders of the blood-brain barrier was recognized. As a highly polar molecule, Gd-DTPA is far too hydrophilic to be able to cross the healthy blood-brain barrier. Changes to the tight junctions, such as those triggered by glioblastomas, enable the paracellular transport of this contrast agent into the interstitium. There it intensifies the contrast through the interaction with the protons of the surrounding water and thus makes the defective areas of the blood-brain barrier visible. Since these blood vessels are responsible for supplying the tumor and are in close proximity to it, the extent of the tumor can be shown.
In the case of an acute ischemic stroke , the disruption of the blood-brain barrier can also be used to make a diagnosis using contrast-enhanced MRI.
By determining the relaxation time , the amount of Gd-DTPA in the interstitium can be quantified.
Other imaging tests
Using radioactively labeled tracers, which normally do not pass the healthy blood-brain barrier, tests can also be carried out to check the function of the blood-brain barrier in humans. In principle, both single-photon emission computed tomography ( SPECT) and positron emission tomography (PET) can be used. For example, an increased uptake of 99m Tc-hexamethylpropylamine oxime (HMPAO) in the brain can be detected in patients with an acute stroke .
With the help of computed tomography (CT) defects of the blood-brain barrier can be quantified by the diffusion of suitable contrast media from the capillaries into the interstitium.
Discovery story
The first proof of the existence of the blood-brain barrier comes from the German chemist Paul Ehrlich . In 1885, when water-soluble acidic vital dyes were injected into the bloodstream of rats, he found that all organs except the brain and spinal cord were stained by the dye.
From this he drew the wrong conclusion in 1904 that a low affinity of the brain tissue for the injected dye was the cause of this finding.
Edwin Goldmann , a former colleague of Paul Ehrlich, injected intravenously in 1909 the di-azo dye trypan blue, which Paul Ehrlich had synthesized for the first time five years earlier . He noticed that the choroid plexus was noticeably stained in contrast to the surrounding brain tissue. In 1913 he injected the same dye directly into the spinal fluid of dogs and rabbits . The entire central nervous system (brain and spinal cord) was stained, but no other organ. Goldmann concluded that the cerebrospinal fluid and the choroid plexus play an important role in transporting nutrients to the central nervous system. Furthermore, he suspected a barrier function for neurotoxic substances.
In 1898 Artur Biedl and Rudolf Kraus carried out experiments with bile acids . When administered systemically into the bloodstream, these compounds were non-toxic. However, when injected into the brain, they were neurotoxic , with reactions up to coma .
In 1900 Max Lewandowsky used potassium hexacyanidoferrate (II) (yellow blood liquor salt) in comparable experiments and came to similar results as Biedl and Kraus. Lewandowsky used the term "blood-brain barrier" for the first time.
In 1890, Charles Smart Roy and the later Nobel Prize winner Charles Scott Sherrington postulated that the brain had an intrinsic mechanism in which the vascular supply corresponds to the local fluctuations in functional activity.
"The brain possesses an intrinsic mechanism by which its vascular supply can be varied locally in correspondence with local variations of functional activity."
Lina Stern , the first female member of the Russian Academy of Sciences , made significant contributions to the research of the blood-brain barrier, which she referred to as the haemato-encephalic barrier in 1921 .
The differentiation between blood-brain and blood-liquor barriers was made by Friedrich Karl Walter and Hugo Spatz in the 1930s . They postulated that the flow of liquor for gas exchange in the central nervous system alone was completely inadequate.
Although Goldmann and Ehrlich's attempts suggested the existence of a barrier between the bloodstream and the central nervous system, there were doubts about its existence until the 1960s. One point of criticism of Goldmann's experiment was that blood and liquor , the two liquids into which the dyes were injected, differ considerably and thus the diffusion behavior and the affinity for the nervous tissue are influenced. Understanding was made even more difficult by the experimental finding that basic azo dyes stained the nerve tissue, i.e. crossed the blood-brain barrier, while the acidic dyes did not. Ulrich Friedemann concluded that the electrochemical properties of the dyes are responsible for this. The cerebral capillaries are permeable to uncharged substances or charged substances that are positive at the pH value of blood and impermeable to acidic substances. This thesis turned out to be inadequate in the period that followed, when a large number of substances were examined for their permeability at the blood-brain barrier. In the following explanatory models, a large number of parameters, such as molar mass, molecule size, binding affinities, dissociation constants, fat solubility, electrical charge and a wide variety of combinations thereof, were discussed.
Today's understanding of the basic structure of the blood-brain barrier is based on electron microscopic images of mouse brains that were carried out at the end of the 1960s. Thomas S. Reese and Morris J. Karnovsky injected horseradish peroxidase intravenously into the test animals in their experiments. When examined with an electron microscope, they found the enzyme only in the lumen of the blood vessels and in micropinocytic vesicles within the endothelial cells. They found no peroxidase outside the endothelium, in the extracellular matrix. They suspected that tight junctions between the endothelial cells prevent the transition to the brain.
literature
- D. Kobiler et al .: Blood-brain Barrier. Verlag Springer, 2001, ISBN 0-306-46708-9 .
- AG De Boer, W. Sutanto: Drug Transport Across the Blood-brain Barrier. CRC Press, 1997, ISBN 90-5702-032-7 .
- WM Pardridge: Introduction to the Blood-brain Barrier. Cambridge University Press, 1998, ISBN 0-521-58124-9 .
- EM Taylor: Efflux Transporters and the Blood-brain Barrier. Nova Publishers, 2005, ISBN 1-59454-625-8 .
- DJ Begley et al .: The Blood-brain Barrier and Drug Delivery to the CNS. Informa Health Care, 2000, ISBN 0-8247-0394-4 .
- E. de Vries, A. Prat: The Blood-brain Barrier and Its Microenvironment. Taylor & Francis, 2005, ISBN 0-8493-9892-4 .
- M. Bradbury: The Concept of a Blood-Brain Barrier. Wiley-Interscience, 1979, ISBN 0-471-99688-2 .
- P. Ramge: Investigations into overcoming the blood-brain barrier with the help of nanoparticles. Shaker Verlag , 1999, ISBN 3-8265-4974-0 .
- P. Brenner: The structure of the blood-brain and blood-liquor barriers. Dissertation, LMU Munich, 2006
- Yasunobu Arima et al .: Regional Neural Activation Defines a Gateway for Autoreactive T Cells to Cross the Blood-Brain Barrier. In: Cell . 148, 2012, pp. 447-457, doi: 10.1016 / j.cell.2012.01.022 .
Web links
- Norman R. Saunders et al .: The rights and wrongs of blood-brain barrier permeability studies: a walk through 100 years of history . In: Frontiers in Neuroscience . tape 8 , December 16, 2014, doi : 10.3389 / fnins.2014.00404 , PMID 25565938 .
Individual evidence
- ↑ a b c d e f g h i j S. Wolf et al .: The blood-brain barrier: a special feature of the cerebral microcirculation system. In: Naturwissenschaften 83, 1996, pp. 302-311. doi: 10.1007 / BF01152211 .
- ^ W. Risau et al .: Immune function of the blood-brain barrier: in complete presentation of protein (auto) antigens by rat brain microvascular endothelium in vitro. In: Journal of Cell Biology 110, 1990, pp. 1757-1766. PMID 1692329 .
- ↑ a b c B. Bauer: In vitro cell culture models of the blood-brain barrier to investigate the permeation and P-glycoprotein interaction of drugs. Dissertation, Ruprecht-Karls-Universität Heidelberg, 2002.
- ↑ a b c d e f g h i j S. Ohtsuki: New aspects of the blood-brain barrier transporters; its physiological roles in the central nervous system. In: Biol Pharm Bull . 27, 2004, pp. 1489-1496. PMID 15467183 (Review).
- ↑ a b c d e M. Bundgaard. NJ Abbott: All vertebrates started out with a glial blood-brain barrier 4-500 million years ago. In: Glia . 56, 2008, pp. 699-708. PMID 18338790 .
- ↑ a b c d e W. M. Pardridge: Molecular biology of the blood-brain barrier. In: Mol Biotechnol , 30, 2005, pp. 57-69. PMID 15805577 (Review).
- ^ JC Lee: Evolution in the concept of the blood-brain barrier phenomen. In: Progress in neuropathology . Volume 1. Verlag Grune and Stratton, 1971, ISBN 0-88167-188-6 , pp. 84-145.
- ↑ a b c M. Pavelka, J. Roth: Functional ultrastructure. Verlag Springer, ISBN 3-211-83563-6 , pp. 234-235.
- ↑ J. Cervos-Navarro: Electron microscopic findings on the capillaries of the cerebral cortex. In: Arch Psychiatr Nervenkr 204, 1963, pp. 484-504. PMID 14082797 .
- ↑ a b c d B. T. Hawkins and TP Davis: The blood-brain barrier / neurovascular unit in health and disease. In: Pharmacol Rev 57, 2005, pp. 173-185. PMID 15914466 (Review).
- ↑ a b c d e f g h i j k S. Nobmann: Isolated brain capillaries as an in vitro model of the blood-brain barrier. Dissertation, Ruprecht-Karls-Universität Heidelberg, 2001.
- ↑ RS el-Bacha, A. Minn: Drug metabolizing enzymes in cerebrovascular endothelial cells afford a metabolic protection to the brain. In: Cell Mol Biol 45, 1999, pp. 15-23. PMID 10099836 (Review).
- ↑ M. Chat et al .: Drug metabolizing enzyme activities and superoxide formation in primary and immortalized rat brain endothelial cells. In: Life Sci 62, 1998, pp. 151-163. PMID 9488113 .
- ↑ A. Minn et al .: Drug metabolizing enzymes in the brain and cerebral microvessels. In: Brain Res Brain Res Rev 116, 1991, pp. 65-82. PMID 1907518 (Review).
- ↑ a b c d Y. Takahura et al .: Blood-brain barrier: transport studies in isolated brain capillaries and in cultured brain endothelial cells. In: Adv Pharmacol 22, 1991, pp. 137-165. PMID 1958501 (Review).
- ↑ S. Meresse et al .: Bovine brain endothelial cells express tight junctions and monoamine oxidase activity in long-term culture. In: J Neurochem 53, 1989, pp. 1363-1371. PMID 2571674 .
- ↑ R. Perrin et al .: Distribution of cytochrom P450 activities towards alkoxyresorufin derivatives in rat brain regions, subcellular fractions and isolated cerebral microvessels. In: Biochem Pharmacol 40, 1990, pp. 2145-2151. PMID 2242042 .
- ↑ R. Bendayan et al .: Functional expression and localization of P-glycoprotein at the blood brain barrier. In: Microsc Res Tech 57, 2002, pp. 365-380. PMID 12112443 (Review).
- ↑ Y. Su, PJ Sinko: Drug delivery across the blood-brain barrier: is why it difficult? how to measure and improve it? In: Expert Opin Drug Deliv 3, 2006, pp. 419-435. PMID 16640501 (Review).
- ↑ a b H. Fischer et al .: Blood – brain barrier permeation: molecular parameters governing passive diffusion. In: J Membr Biol 165, 1998, pp. 201-211. PMID 9767674 .
- ↑ a b U. Fagerholm: The highly permeable blood-brain barrier: an evaluation of current opinions about brain uptake capacity. In: Drug Discovery Today 12, 2007, pp. 1076-1082. PMID 18061888 (Review).
- ↑ B. Nico et al .: Role of aquaporin-4 water channel in the development and integrity of the blood-brain barrier. In: J Cell Sci 114, 2001, pp. 1297-1307. PMID 11256996 .
- ↑ a b A. M. Butt et al .: Electrical resistance across the blood-brain barrier in anaesthetized rats: a developmental study. In: J Physiol 429, 1990, pp. 47-62. PMID 2277354 .
- ^ P. Claude, DA Goodenough: Fracture faces of zonae occludentes from tight and leaky epithelia. In: J Cell Biol , 58, 1973, pp. 390-400. PMID 4199658 .
- ^ H. Wolburg et al .: Modulation of tight junction structure in blood-brain barrier endothelial cells. Effects of tissue culture, second messengers and cocultured astrocytes. In: J Cell Sci 107, 1994, pp. 1347-1357. PMID 7929640 .
- ↑ a b H. B. Newton: Advances in strategies to improve drug delivery to brain tumors. In: Expert Rev Neurother 6, 2006, pp. 1495-1509. PMID 17078789 (Review).
- ↑ JL Madara: Tight junction dynamics: is paracellular transport regulated? In: Cell 53, 1988, pp. 497-498. PMID 3286009 .
- ^ HC Bauer et al .: Proteins of the tight junctions in the blood-brain barrier. In: Blood-spinal Cord and Brain Barriers in Health and Disease . Verlag Elsevier, 2004, pp. 1-10.
- ↑ R. Cecchell et al .: Modeling of the blood-brain barrier in drug discovery and development. In: Nat Rev Drug Discov , 6, 2007, pp. 650-661. PMID 17667956 (Review).
- ^ K. Matter, MS Balda: Holey barrier: claudins and the regulation of brain endothelial permeability. In: J Cell Biol , 161, 2003, pp. 459-460. PMID 12743096 (Review).
- ↑ T. Nitta et al .: Size-selective loosening of the blood-brain barrier in claudin-5-deficient mice. In: J Cell Biol , 161, 2003, pp. 653-660. PMID 12743111 .
- ^ A b P. Dore-Duffy: Pericytes: pluripotent cells of the blood brain barrier. In: Curr Pharm Des 14, 2008, pp. 1581-1593. PMID 18673199 (Review).
- ^ R. Balabanov, P. Dore-Duffy: Role of the CNS microvascular pericyte in the blood-brain barrier. In: J Neurosci Res 53, 1998, pp. 637-644. PMID 9753191 (Review).
- ↑ a b c d H.K. Rucker et al .: Cellular mechanisms of CNS pericytes. In: Brain Res Bull 51, 2000, pp. 363-369. PMID 10715555 (Review).
- ^ PA D'Amore: Culture and Study of Pericytes. In: Cell Culture Techniques in Heart and Vessel Research . Verlag Springer, 1990, ISBN 3-540-51934-3 , p. 299.
- ↑ NJ Abbott: Neurobiology: Glia and the blood-brain barrier. In: Nature , 325, 1987, p. 195. PMID 3808015 .
- ^ CH Lai, KH Kuo: The critical component to establish in vitro BBB model: Pericyte. In: Brain Res Brain Res Rev , 50, 2005, pp. 258-265. PMID 16199092 (Review).
- ↑ a b D. Shepro, NM Morel: physiology pericyte. In: FASEB J , 7, 1993, pp. 1031-1038. PMID 8370472 (Review).
- ↑ K. Fujimoto: Pericyte-endothelial gap junctions in developing rat cerebral capillaries: A fine structural study. In: Anat Rec 242, 1995, pp. 562-565. PMID 7486026 .
- ↑ L. Diaz-Flores: Microvascular pericytes: A review of their morphological and functional characteristics. In: Histol Histopath 6, 1991, pp. 269-286. PMID 1802127 (Review).
- ^ DE Sims: Recent advances in pericyte biology - Implications for health and disease. In: Can J Cardiol 7, 1991, pp. 431-443. PMID 1768982 (Review).
- ↑ a b c D. E. Sims: Diversity within pericytes. In: Clin Exp Pharmacol Physiol 27, 2000, pp. 842-846. PMID 11022980 (review).
- ↑ IM Herman, PA D'Amore: Microvascular pericytes contain muscle and nonmuscle actins. In: J Cell Biol , 101, 1985, pp. 43-52. PMID 3891763 .
- ↑ KK Hirschi, PA D'Amore: Pericytes in the microvasculature. In: Cardiovasc Res , 32, 1996, pp. 687-698. PMID 8915187 (review).
- ↑ M. Mato et al .: Evidence for the possible function of the fluorescent granular perithelial cells in brain as scavengers of high-molecular-weight waste products. In: Experientia 40, 1984, pp. 399-402. PMID 6325229 .
- ↑ R. Balabanov et al .: CNS microvascular pericytes express macrophage-like function, cell surface integrin alphaM, and macrophage marker ED-2. In: Microvasc Res 52, 1996, pp. 127-142. PMID 8901442 .
- ↑ WF Hickey and H. Kimura: Perivascular microglial cells of the CNS are bone marrow-derived and present antigen in vivo. In: Science 239, 1988, pp. 290-292. PMID 3276004 .
- ↑ Z. Fabry et al .: Differential activation of Th1 and Th2 CD4 + cells by murine brain microvessel endothelial cells and smooth muscle / pericytes. In: J Immunol 151, 1993, pp. 38-47. PMID 8100844 .
- ↑ D. Krause et al .: Cerebral pericytes - a second line of defense in controlling blood-brain barrier peptide metabolism. In: Adv Exp Med Biol 331, 1993, pp. 149-152. PMID 8101424 .
- ^ WE Thomas: Brain macrophages: on the role of pericytes and perivascular cells. In: Brain Res Brain Res Rev 31, 1999, pp. 42-57. PMID 10611494 (Review).
- ↑ C. Iadecola: Neurovascular regulation in the normal brain and in Alzheimer's disease. In: Nat Rev Neurosci 5, 2004, pp. 347-360. PMID 15100718 (Review).
- ^ B. Engelhardt: Development of the blood-brain barrier. In: Cell Tissue Res 314, 2003, pp. 119-129. PMID 12955493 (Review).
- ^ CE Johanson: Permeability and vascularity of the developing brain: cerebellum vs. cerebral cortex. In: Brain Res 190, 1980, pp. 3-16. PMID 6769537 .
- ^ J. Neuhaus et al .: Induction of blood-brain barrier characteristics in bovine brain endothelial cells by rat astroglial cells in transfilter coculture. In: Ann NY Acad Sci 633, 1991, pp. 578-580. PMID 1789585 .
- ↑ PA Stewart, MJ Wiley: Developing nervous tissue induces formation of blood-brain barrier characteristics in invading endothelial cells: a study using quail-chick transplantation chimeras. In: Dev Biol , 84, 1981, pp. 183-192. PMID 7250491 .
- ^ TJ Raub et al .: Permeability of bovine brain microvessel endothelial cells in vitro: barrier tightening by a factor released from astroglioma cells. In: Exp Cell Res , 199, 1992, pp. 330-340. PMID 1347502 .
- ↑ a b c d N. J. Abbott: Astrocyte-endothelial interactions and blood-brain barrier permeability. In: J Anat 200, 2002, pp. 629-638. PMID 12162730 (Review).
- ↑ a b O. B. Paulson, EA Newman: Does the release of potassium from astrocyte endfeet regulate cerebral blood flow? In: Science , 237, 1987, pp. 896-898. PMID 3616619 .
- ↑ NJ Abbott et al .: Astrocyte-endothelial interactions at the blood-brain barrier. In: Nat Rev Neurosci , 7, 2006, pp. 41-53. PMID 16371949 (Review).
- ↑ I. Björkhem, S. Meaney: Brain Cholesterol: Long Secret Life Behind a barrier. In: Arterioscler Thromb Vasc Biol , 24, 2004, pp. 806-815. PMID 14764421 (Review).
- ↑ HM Duvernoy, PY Risold: The circumventricular organs: of atlas of comparative anatomy and vascularization. In: Brain Res Rev 56, 2007, pp. 119-147. PMID 17659349 (Review).
- ↑ C. Lohmann: The blood-brain barrier in vitro: regulation of permeability by matrix metalloproteases. Dissertation, Westfälische Wilhelms-Universität Münster, 2003.
- ↑ World Cup Pardridge: Peptide Drug Delivery to the Brain. Raven Press, 1991, ISBN 0-88167-793-0 , p. 123.
- ↑ WL Chiou, A. Barve: linear correlation of the fraction of oral dose absorbed of 64 drugs between humans and rats. In: Pharm Res , 15, 1998, pp. 1792-1795. PMID 9834005 .
- ^ JT Goodwin and DE Clark: In silico predictions of blood-brain barrier penetration: considerations to "keep in mind". In: J Pharmacol Exp Ther 315, 2005, pp. 477-483. PMID 15919767 (Review).
- ^ SL Lindstedt and PJ Schaeffer: Use of allometry in predicting anatomical and physiological parameters of mammals. In: Lab Anim 36, 2002, pp. 1-19. PMID 11833526 .
- ↑ RW Leggett, LR Williams: A proposed blood circulation model for reference man. In: Health Phys , 69, 1995, pp. 187-201. PMID 7622365 .
- ^ S. Willmann et al .: A physiological model for the estimation of the fraction dose absorbed in humans. In: J Med Chem 47, 2004, pp. 4022-4031. PMID 15267240 .
- ↑ U. Fagerholm et al .: Comparison between permeability coefficients in rat and human jejunum. In: Pharm Res , 13, 1996, pp. 1336-1342. PMID 8893271 .
- ↑ RW Leggett, LR Williams: Suggested reference values for regional blood volumes in humans. In: Health Phys , 60, 1991, pp. 139-154. PMID 1989937 .
- ↑ GB Wislocki: Experimental studies on fetal absorption. I. The vitally stained fetus. In: Contrib Embryol Carnegie Inst , 5, 1920, pp. 45-52.
- ↑ S. Wakai, N. Hirokawa: Development of the blood-brain barrier to horseradish peroxidase in the chick embryo. In: Cell Tissue Res , 195, 1979, pp. 195-203. PMID 737715 .
- ^ W. Risau et al .: Differentiation-dependent expression of proteins in brain endothelium during development of the blood-brain barrier. In: Dev Biol 117, 1986, pp. 537-545. PMID 2875908 .
- ↑ ML Reynolds et al .: Intracranial haemorrhage in the preterm sheep fetus. In: Early Hum Dev 3, 1979, pp. 163-186. PMID 575326 .
- ↑ L. Stern and R. Peyrot: Le fonctionnement de la barrière hémato-éncephalique aux divers stades de développement chez les diverses espèces animales. In: Compte Rendu des Societe de Biologie (Paris) 96, 1927, pp. 1124-1126.
- ↑ L. Stern et al .: Le fonctionnement de la barrière hémato-éncephalique chez les nouveau-nés. In: Compte Rendu Soc Biol 100, 1929, pp. 231-233.
- ↑ a b N. R. Saunders et al .: Barrier mechanisms in the brain, II. Immature brain. In: Clin Exp Pharmacol Physiol 26, 1999, pp. 85-91. PMID 10065326 (Review).
- ^ NR Saunders: Development of the blood-brain barrier to macromolecules. In: The Fluids and Barriers of the Eye and Brain Editor: MB Segal, Verlag MacMillan, 1991, ISBN 0-8493-7707-2 , pp. 128-155.
- ↑ U. Schumacher, K. Møllgård: The multidrug resistance P-glycoprotein (Pgp, MDR1) is an early marker of blood-brain barrier development in the microvessels of the developing human brain. In: Histochem Cell Biol , 108, 1997, pp 179-182. PMID 9272437 .
- ↑ KM Dziegielewska et al .: Studies of the development of brain barrier systems to lipid insoluble molecules in fetal sheep. In: J Physiol , 292, 1979, pp. 207-231. PMID 490348 .
- ^ RK Ferguson, DM Woodbury: Penetration of 14C-inulin and 14C-sucrose into brain, cerebrospinal fluid and skeletal muscle of developing rats. In: Exp Brain Res , 7, 1969, pp. 181-194. PMID 5795246 .
- ^ MD Habgood et al .: The nature of the decrease in blood – cerebrospinal fluid barrier exchange during postnatal brain development in the rat. In: J Physiol 468, 1993, pp. 73-83. PMID 8254533 .
- ^ CE Johanson: Ontogeny of the blood-brain barrier. In: Implications of the Blood-Brain Barrier and Its Manipulation Editor: EA Neuwelt, Plenum Press, 1989, pp. 157-198.
- ↑ LD Braun et al .: Newborn rabbit blood-brain barrier is selectively permeable and differs substantially from the adult. In: J Neurochem 34, 1980, pp. 147-152. PMID 7452231 .
- ^ EM Cornford et al .: Developmental modulations of blood-brain barrier permeability as an indicator of changing nutritional requirements in the brain. In: Pediatr Res 16, 1982, pp. 324-328. PMID 7079003 .
- ↑ DP Brenton, RM Gardiner: Transport of L-phenylalanine and related amino acids at the ovine blood-brain barrier. In: J Physiol , 402, 1988, pp. 497-514. PMID 3236248 .
- ↑ HJL Frank et al .: Enhanced insulin binding to blood-brain barrier in vivo and to brain microvessels in vitro in newborn rabbits. In: Diabetes , 34, 1985, pp. 728-733. PMID 3894116 .
- ^ NR Saunders: Barriers in the immature brain. In: Cell Mol Neurobiol , 20, 2000, pp. 29-40. PMID 10690500 (Review).
- ↑ NJ Abbott, M. Bundgaard: Electron-dense tracer evidence for a blood-brain barrier in the cuttlefish, Sepia officinalis. In: J Neurocytol , 21, 1992, pp. 276-294. PMID 1588347 .
- ↑ NJ Abbott, Y. Pichon: The glial blood-brain barrier of crustacea and cephalopods: a review. In: J Physiol (Paris) , 82, 1987, pp. 304-313. PMID 3332691 (Review).
- ↑ a b N. J. Abbott: Dynamics of CNS barriers: evolution, differentiation, and modulation. In: Cell Mol Neurobiol 25, 2005, pp. 5-23. PMID 15962506 (Review).
- ↑ NJ Abbott: Comparative physiology of the blood-brain barrier. In: Physiology and pharmacology of the bloodbrain barrier Editor: MWB Bradbury, Springer-Verlag, 1992, ISBN 0-387-54492-5 , pp. 371-396.
- ↑ T. Stork et al .: Organization and function of the blood-brain barrier in Drosophila. In: J Neurosci 28, 2008, pp. 587-597. PMID 18199760 .
- ↑ J. Fraher and E. Cheong: glial-Schwann cell specialisations at the central-peripheral nervous system of a transition cyclostome: study on ultra-structural. In: Acta Anat 154, 1995, pp. 300-314. PMID 8773716 .
- ↑ NJ Abbott et al .: Comparative physiology of the blood-brain barrier. In: The blood-brain barrier in health and disease. Editors: AJ Suckling et al., Ellis Horwood Verlag, 1986, pp. 52-72.
- ↑ F. Miragall et al .: Expression of the tight junction protein ZO-1 in the olfactory system: Presence of ZO-1 on olfactory sensory neurons and glial cells. In: J Comp Neurol 341, 1994, pp. 433-448. PMID 8201022 .
- ↑ a b c N. Hettenbach: Influence of chronic electromagnetic exposure to mobile phone radiation (GSM and UMTS) on the integrity of the blood-brain barrier in rats. Dissertation, Ludwig Maximilians University Munich, 2008.
- ^ SI Rapoport: Blood-brain Barrier in Physiology and Medicine. Raven Press, 1976, ISBN 0-89004-079-6 .
- ^ A b c M. Fromm: Physiology of humans - transport in membranes and epithelia. (Editor: RF Schmidt, F. Lang), Verlag Springer, ISBN 978-3-540-32908-4 , pp. 41-54.
- ↑ I. Sauer: Apolipoprotein E derived peptides as vectors for overcoming the blood-brain barrier. Dissertation FU Berlin, 2004. urn : nbn: de: kobv: 188-2004003141
- ↑ a b R. D. Egleton, TP Davis: Development of neuropeptide drugs did cross the blood-brain barrier. In: NeuroRx 2, 2005, pp. 44-53. PMID 15717056 (Review).
- ^ WH Oldendorf: Lipid solubility and drug penetration of the blood-brain barrier. In: Proc Soc Exp Biol Med 147, 1974, pp. 813-816. PMID 4445171 .
- ↑ a b c R. Kaliszan and M. Markuszewski: Brain / blood distribution Described by a combination of partition coefficient and molecular mass. In: International Journal of Pharmaceutics 145, 1996, pp. 9-16. doi: 10.1016 / S0378-5173 (96) 04712-6 .
- ^ H. Träuble: Carriers and specificity in membranes. 3. Carrier-facilitated transport. Kinks as carriers in membranes. In: Neurosci Res Program Bull 9, 1971, pp. 361-372. PMID 5164654 .
- ^ H. Träuble: Phase changes in lipids. Possible switching processes in biological membranes. In: Naturwissenschaften 58, 1971, pp. 277–284. PMID 4935458 (Review).
- ↑ O. Vostowsky: Chemistry of Natural Products - lipoproteins and membranes. University of Erlangen, 2005, p. 42.
- ^ W. Hoppe, RD Bauer: Biophysik. Verlag Birkhäuser, 1982, ISBN 0-387-11335-5 , pp. 447-448.
- ^ A. Seelig and J. Seelig: The dynamic structure of fatty acyl chains in a phospholipid bilayer measured by deuterium magnetic resonance. In: Biochemistry 13, 1974, pp. 4839-4845. PMID 4371820 .
- ↑ A. Elbert: The permeation of small polar molecules through phospholipid model membranes. Dissertation, University of Kaiserslautern, 1999. urn : nbn: de: bsz: 386-kluedo-12797
- ^ VA Levin: Relationship of octanol / water partition coefficient and molecular weight to rat brain capillary permeability. In: J Med Chem 23, 1980, pp. 682-684. PMID 7392035 .
- ↑ A. Seelig et al .: A method to determine the ability of drugs to diffuse throught the blood-brain barrier. In: PNAS 91, 1994, pp. 68-72. PMID 8278409 .
- ↑ a b G. A. Dhopeshwarkar and JF Mead: Uptake and transport of fatty acids into the brain and the role of the blood-brain barrier system. In: Adv Lipid Res 11, 1973, pp. 109-142. PMID 4608446 (Review).
- ↑ G. Gerebtzoff, A. Seelig: In silico prediction of blood-brain barrier permeation using the calculated molecular cross-sectional area as main parameter. In: AJ Chem Inf Model , 46, 2006, pp. 2638-2650. PMID 17125204 .
- ↑ a b W. W. Pardridge: The blood-brain barrier: bottleneck in brain drug development. In: NeuroRx 2, 2005, pp. 3-14. PMID 15717053 (Review).
- ^ A b W. H. Oldendorf: Measurement of brain uptake of radiolabeled substances using a tritiated water internal standard. In: Brain Res , 24, 1970, pp. 372-376. PMID 5490302 .
- ↑ D. Dolman et al .: Induction of aquaporin 1 but not aquaporin 4 messenger RNA in rat primary brain microvessel endothelial cells in culture. In: J Neurochem 93, 2005, pp. 825-833. PMID 15857386 .
- ^ O. Bloch and GT Manley: The role of aquaporin-4 in cerebral water transport and edema. In: Neurosurg Focus 22, 2007, p. E3 PMID 17613234 (Review).
- ↑ AS Verkman: More than just water channels: unexpected cellular roles of aquaporins. In: J Cell Sci 118, 2005, pp. 3225-3232. PMID 16079275 (Review).
- ↑ a b J. Badaut et al .: Aquaporins in the brain: from aqueduct to "multi-duct". In: Metab Brain Dis 22, 2007, pp. 251-263. PMID 17701333 (Review).
- ↑ Farrell et al .: Blood-brain barrier glucose transporter is asymmetrically distributed on brain capillary endothelial lumenal and ablumenal membranes: An electron microscopic immunogold study . In: Proc. Natl. Acad. Sci. USA , Vol. 88, 1991, pp. 5779-5783.
- ↑ A. Dahlin, J. Royall, JG Hohmann, J. Wang: Expression Profiling of the Solute Carrier (SLC) Gene Family in the Mouse Brain. In: J. Pharmacol. Exp. Ther. 329, 2009, pp. 558-570 PMID 19179540 PMC 267287 (free full text).
- ↑ D. Agus et al .: Vitamin C crosses the blood-brain barrier in the oxidized form through the glucose transporters. In: J Clin Invest 100, 1997, pp. 2842-2848. PMID 9389750 .
- ^ EM Cornford and S. Hyman: Blood-brain barrier permeability to small and large molecules. In: Advanced Drug Delivery Reviews 36, 1999, pp. 145-163. PMID 10837713 (Review).
- ↑ BV Zlokovic et al .: Transport of leucine-enkephalin across the blood-brain barrier in the perfused guinea pig brain. In: J Neurochem 49, 1987, pp. 310-315. PMID 3585338 .
- ↑ BV Zlokovic et al .: Kinetic analysis of leucine-enkephalin cellular uptake at the luminal side of the blood-brain barrier of an in situ perfused guinea-pig brain. In: J Neurochem 53, 1989, pp. 1333-1340. PMID 2795003 .
- ↑ BV Zlokovic et al .: Kinetics of arginine-vasopressin uptake at the bloodbrain barrier. In: Biochim Biophys Acta 1025, 1990, pp. 191-198. PMID 2364078 .
- ^ SA Thomas et al .: The entry of [D-penicillamine2,5] enkephalin into the central nervous system: saturation kinetics and specificity. In: J Pharmacol Exp Ther 280, 1997, pp. 1235-1240. PMID 9067309 .
- ↑ VV Rao et al .: Choroid plexus epithelial expression of MDR1 P glycoprotein and multidrug resistance-associated protein contribute to the blood-cerebrospinal-fluid drug-permeability barrier. In: PNAS 96, 1999, pp. 3900-3905. PMID 10097135 .
- ↑ F. Thiebaut et al .: Immunohistochemical localization in normal tissues of different epitopes in the multidrug transport protein P170: evidence for localization in brain capillaries . In: J Histochem Cytochem 37, 1989, pp. 159-164, PMID 2463300 .
- ↑ DJ Begley: ABC transporters and the blood-brain barrier. In: Curr Pharm Des 10, 2004, pp. 1295-1312. PMID 15134482 (Review).
- ↑ S. Seetharaman et al .: Multidrug resistance-related transport proteins in isolated human brain microvessels and in cells cultured from these isolates. In: J Neurochem 70, 1998, pp. 1151-1159. PMID 9489736 .
- ↑ a b H. C. Cooray et al .: Localization of breast cancer resistance protein in microvessel endothelium of human brain. In: Neuroreport 13, 2002, pp. 2059-2063. PMID 12438926 .
- ↑ T. Eisenblätter and HJ Galla: A new multidrug resistance protein at the blood-brain barrier. In: Biochem Biophys Res Commun 293, 2002, pp. 1273-1278. PMID 12054514 .
- ^ Y. Tanaka et al .: Ultrastructural localization of P-glycoprotein on capillary endothelial cells in human gliomas. In: Virchows Arch 425, 1994, pp. 133-138. PMID 7952498 .
- ↑ EC de Lange: Potential role of ABC transporters as a detoxification system at the blood-CSF barrier. In: Adv Drug Deliv Rev 56, 2004, pp. 1793-1809. PMID 15381334 (Review).
- ↑ H. Wolosker et al .: Neurobiology through the looking-glass: D-serine as a new glial-derived transmitter. In: Neurochem Int , 41, 2002, pp. 327-332. PMID 12176074 (Review).
- ↑ CF Zorumski and JW Olney: Excitotoxic neuronal damage and neuropsychiatric disorders In: Pharmacol Ther 59, 1993, pp. 145-162. PMID 7904075 (Review).
- ↑ K. Hosoya et al .: Blood-brain barrier produces significant efflux of L-aspartic acid but not D-aspartic acid: in vivo evidence using the brain efflux index method. In: J Neurochem , 73, 1999, pp. 1206-1211. PMID 10461913 .
- ↑ M. Palacin et al .: Molecular biology of mammalian plasma membrane amino acid transporters. In: Physiol Rev , 78, 1998, pp. 969-1054. PMID 9790568 (Review).
- ↑ W. Löscher and H. Potschka: Blood-brain barrier active efflux transporters: ATP-binding cassette gene family. In: NeuroRx , 2, 2005, pp. 86-98. PMID 15717060 (Review).
- ↑ DM Tishler et al .: MDR1 gene expression in brain of patients with medically intractable epilepsy. In: Epilepsia , 36, 1995, pp. 1-6. PMID 8001500 .
- ↑ H. Kusuhara et al .: Molecular cloning and characterization of a new multispecific organic anion transporter from rat brain. In: J Biol Chem , 274, 1999, pp. 13675-13680. PMID 10224140 .
- ↑ B. Gao et al .: Localization of the organic anion transporting polypeptide 2 (Oatp2) in capillary endothelium and choroid plexus epithelium of rat brain. ( Memento of September 5, 2008 in the Internet Archive ) In: J Histochem Cytochem , 47, 1999, pp. 1255-1264. PMID 10490454 .
- ^ LZ Bito: Blood-Brain Barrier: Evidence for Active Cation Transport between Blood and the Extraceliular Fluid of Brain. In: Science 165, 1969, pp. 81-83. PMID 17840696 .
- ↑ Y. Kido et al .: Functional relevance of carnitine transporter OCTN2 to brain distribution of L-carnitine and acetyl-L-carnitine across the blood-brain barrier. In: J Neurochem 79, 2001, pp. 959-969. PMID 11739607 .
- ^ RL Roberts et al .: Receptor-mediated endocytosis of transferrin at the blood-brain barrier. In: J Cell Sci 104, 1993, pp. 521-532. PMID 8505377 .
- ↑ B. Dehouck et al .: Upregulation of the low density lipoprotein receptor at the blood-brain barrier: intercommunications between brain capillary endothelial cells and astrocytes. In: J Cell Biol 126, 1994, pp. 465-473. PMID 8034745 .
- ↑ KR Duffy et al .: Human blood-brain barrier insulin-like growth factor receptor. In: Metabolism 37, 1988, pp. 136-140. PMID 2963191 .
- ↑ MW Smith and M. Gumbleton: Endocytosis at the blood-brain barrier: from basic understanding to drug delivery strategies. In: J Drug Target 14, 2006, pp. 191-214. PMID 16777679 (Review).
- ↑ F. Hervé et al .: CNS delivery via adsorptive transcytosis. In: AAPS J 10, 2008, pp. 455-472. PMID 18726697 (Review).
- ↑ JM Scherr man: Drug delivery to brain via the blood-brain barrier. In: Vascul Pharmacol 38, 2002, pp. 349-354. PMID 12529929 (Review).
- ↑ I. Tamai et al .: Structure-internalization relationship for adsorptive-mediated endocytosis of basic peptides at the blood-brain barrier. In: J Pharmacol Exp Ther 280, 1997, pp 410-415. PMID 8996222 .
- ↑ a b c d e f g h i j k l m n W. M. Pardridge: Blood-brain barrier delivery. In: Drug Discov Today 12, 2007, pp. 54-61. PMID 17198973 .
- ↑ a b c d e f H. Bronger et al .: ABCC drug efflux pumps and organic anion uptake transporters in human gliomas and the blood-tumor barrier. In: Cancer Res 65, 2005, pp. 11419-11428. PMID 16357150 .
- ^ H. Kusuhara and Y. Sugiyama: Active efflux across the blood-brain barrier: role of the solute carrier family. In: NeuroRx 2, 2005, pp. 73-85. PMID 15717059 (Review).
- ↑ JM Scherrmann: Expression and function of multidrug resistance transporters at the blood-brain barriers. In: Expert Opin Drug Metab Toxicol 1, 2005, pp. 233-246. PMID 16922639 (Review).
- ↑ N. Bodor and P. Buchwald: Recent advances in the brain targeting of neuropharmaceuticals by chemical delivery systems. In: Adv Drug Deliv Rev 36, 1999, pp. 229-254. PMID 10837718 (Review).
- ↑ a b c d e f U. Bickel: How to measure drug transport across the blood-brain barrier. In: NeuroRx 2, 2005, pp. 15-26. PMID 15717054 (Review).
- ↑ a b J. window Wheeler, L. Wei: Measuring local cerebral capillary permeability-surface area products by quantitative autoradiography. In: Introduction to the Blood-brain Barrier WM Pardridge (Editor), Cambridge University Press, 1998, ISBN 0-521-58124-9 , pp. 122-132.
- ^ C. Crone and DG Levitt: Capillary permeability to small solutes. In: Handbook of Physiology American Physiological Society, 1984, pp. 375-409.
- ↑ H. Pötzsch: Perfusion computed tomographic measurement parameters in the context of clinicopathological properties of squamous cell carcinoma of the oral cavity and the oropharynx. Dissertation, FU Berlin, 2007.
- ↑ AM Peters: Fundamentals of tracer kinetics for radiologists. In: Br J Radiol 71, 1998, pp. 1116-1129. PMID 10434905 (Review).
- ↑ F. Lasbennes et al .: Capacity for energy metabolism in microvessels isolated from rat brain. In: Neurochem Res 9, 1984, pp. 1-10. PMID 6325972 .
- ↑ J. Huwyler and WM Pardridge: Examination of blood-brain barrier transferrin receptor by confocal fluorescent microscopy of unfixed isolated rat brain capillaries. In: J Neurochem 70, 1998, pp. 883-886. PMID 9453586 .
- ↑ DS Miller et al .: Xenobiotic transport across isolated brain microvessels studied by confocal microscopy. In: Mol Pharmacol 58, 2000, pp. 1357-1367. PMID 11093774 .
- ↑ a b M. Gumbleton et al .: Progress and limitations in the use of in vitro cell cultures to serve as a permeability screen for the blood-brain barrier. In: J Pharm Sci 90, 2001, pp. 1681-1698. PMID 11745727 (Review).
- ^ A. Reichel et al .: An overview of in vitro techniques for blood-brain barrier studies. In: Methods Mol Med 89, 2003, pp. 307-324. PMID 12958429 .
- ↑ R. Cecchelli et al .: In vitro model for evaluating drug transport across the blood-brain barrier. In: Adv Drug Deliv Rev 36, 1999, pp. 165-178. PMID 10837714 .
- ↑ D. Hoheisel et al .: Hydrocortisone reinforces the blood-brain properties in a serum free cell culture system. In: Biochem Biophys Res Commun 247, 1998, pp. 312-315. PMID 9679029 .
- ↑ SB Raymond et al .: Ultrasound Enhanced Delivery of Molecular Imaging and Therapeutic Agents in Alzheimer's Disease Mouse Models. In: PLoS ONE 3, 2008, e2175. PMID 18478109 .
- ↑ JD Fenstermacher et al .: Methods for quantifying the transport of drugs across the blood-brain system. In: Pharmacol Ther 14, 1981, pp. 217-248. PMID 7031708 .
- ^ G. Molema, DKF Meijer: Drug Targeting: Organ-specific Strategies. Wiley-VCH, 2001, ISBN 3-527-29989-0 .
- ↑ JE Hardebo, B. Nilsson: Estimation of cerebral extraction of circulating compounds by the brain uptake index method: influence of circulation time, volume injection, and cerebral blood flow. In: Acta Physiol Scand 107, 1979, pp. 153-159. PMID 525379 .
- ↑ Y. Moriki et al .: Involvement of P-glycoprotein in blood-brain barrier transport of pentazocine in rats using brain uptake index method. In: Biol Pharm Bull 27, 2004, pp. 932-935. PMID 15187451 .
- ↑ LJ Murphey and GD Olsen: Diffusion of morphine-6-beta-D-glucuronide into the neonatal guinea pig brain during drug-induced respiratory depression. In: J Pharmacol Exp Ther 271, 1994, pp. 118-124. PMID 7965704 .
- ^ WH Oldendorf et al .: Blood-brain barrier penetration abolished by N-methyl quaternization of nicotine. In: PNAS 90, 1993, pp. 307-311. PMID 8419935 .
- ↑ D. Magnani: Investigations of the binding of foreign substances in permanent keratinocyte and melanocyte cell lines as well as the suitability of primary cultures from human hair follicles for in vitro measurement of the foreign substance uptake in hair. Dissertation, Carl von Ossietzky University of Oldenburg, 2005. urn : nbn: de: gbv: 715-oops-1151
- ↑ a b E. M. Cornford et al .: Comparison of lipid-mediated blood-brain-barrier penetrability in neonates and adults. In: Am J Physiol-Cell Physiol 243, 1982, pp. 161C-168C. PMID 7114247 .
- ↑ A. Kakee et al .: Organic anion transport system acting as an efflux pump at the blood-brain barrier: demonstration by a newly developed brain efflux index (BEI) method. In: Proc Int Symp Control Release Bioact Mater 22, 1995, pp. 16-18.
- ↑ a b I. Tamai, A. Tsuji: Drug delivery through the blood-brain barrier. In: Adv Drug Deliv Rev 19, 1996, pp. 401-424. doi: 10.1016 / 0169-409X (96) 00011-7 (Review).
- ↑ BV Zlokovic et al .: Measurement of solute transport in the perfused guinea pig brain. Method application to N-methyl-alpha-aminoisobutyric acid. In: J Neurochem 46, 1986, pp. 1444-1451. PMID 3083044 .
- ↑ D. Triguero et al .: Capillary depletion method for quantification of blood-brain barrier transport of circulating peptides and plasma proteins. In: J Neurochem 54, 1990, pp. 1882-1888. PMID 2338547 .
- ↑ C. Crone: The permeability of capillaries in various organs as determined by use of the 'Indicator Diffusion' method. In: Acta Physiol Scand 58, 1963 pp. 292-305. PMID 14078649 .
- ↑ JB van Bree et al .: Drug transport across the blood-brain barrier, II. Experimental techniques to study drug transport. In: Pharma Weekbl Sci 14, 1992, pp. 338-348. PMID 1475172 (Review).
- ↑ L. Sokoloff et al .: The [14C] deoxyglucose method for the measurement of local cerebral glucose utilization: theory, procedure, and normal values in the conscious and anesthetized albino rat. In: J Neurochem 28, 1977, pp. 897-916. PMID 864466 .
- ↑ JD Fenstermacher, L. Wei: Measuring local cerebral capillary permeability-surface area products by quantitative autoradiography. In: Introduction to the blood-brain barrier: methodology, biology and pathology Cambridge University Press, 1998, ISBN 0-521-58124-9 , pp. 122-132.
- ↑ a b E. CM de Lange et al .: The use of intacerebral microdialysis to determine the changes in blood-brain barrier transport characteristics. In: Pharm Res 12, 1995, pp. 129-133. PMID 7724474 .
- ↑ ECM de Lange et al .: Critical factors of intracerebral microdialysis as a technique to determine the pharmacokinetics of drugs in rat brain. In: Brain Res 666, 1994, pp. 1-8. PMID 7889356 .
- ↑ a b E. CM de Lange et al .: Methodological considerations of intracerebral microdialysis in pharmacokinetic studies on drug transport across the blood-brain barrier. In: Brain Res Brain Res Rev 25, 1997, pp. 27-49. PMID 9370049 (Review).
- ↑ ECM de Lange et al .: BBB transport and P-glycoprotein functionality using MDR1A (- / -) and wild-type mice. Total brain versus microdialysis concentration profiles of rhodamine-123. In: Pharm Res 15, 1998, pp. 1657-1665. PMID 9833984 .
- ↑ T. Terasaki et al .: In vivo transport of a dynorphin-like analgesic peptide, E 2078, through the blood-brain barrier: an application of microdialysis. In: J Pharmacol Exp Ther 251, 1991, pp. 815-820. PMID 1681528 .
- ↑ C. Berger et al .: Cerebral microdialysis in stroke. In: Der Nervenarzt 75, 2004, pp. 113–123. PMID 14770280 .
- ↑ G. Paterno: Die cerebral microdialysis (PDF; 186 kB) a practice report, September 2001.
- ↑ a b S. Syvänen et al .: Species differences in blood-brain barrier transport of three PET radioligands with emphasis on P-glycoprotein transport. In: Drug Metab Dispos 37, 2009, pp. 635-643. PMID 19047468 .
- ↑ a b J. Bart et al .: New positron emission tomography tracer (11) C-carvedilol reveals P-glycoprotein modulation kinetics. In: Br J Pharmacol 145, 2005, pp. 1045-1051. PMID 15951832 .
- ^ NH Hendrikse et al .: A new in vivo method to study P-Glycoprotein transport in tumors and the blood-brain barrier. In: Cancer Res 59, 1999, pp. 2411-2416. PMID 10344751 .
- ↑ SS Zoghbi et al .: 11C-loperamide and its N-desmethyl radiometabolite are avid substrates for brain permeability-glycoprotein efflux. In: J Nucl Med 49, 2008, pp. 649-656. PMID 18344435 .
- ^ V. Josserand et al .: Evaluation of Drug Penetration into the Brain: A Double Study by in Vivo Imaging with Positron Emission Tomography and Using an in Vitro Model of the Human Blood-Brain Barrier. In: J Pharmacol Exp Ther 316, 2006, pp. 79-86. PMID 16210395 .
- ↑ D. Matsuzawa et al .: Negative Correlation between Brain Glutathione Level and Negative Symptoms in Schizophrenia: A 3T 1H-MRS Study. In: PLoS ONE 3, 2008, e1944. PMID 18398470 .
- ↑ M. Bartels et al .: 19F Nuclear Magnetic Resonance Spectroscopy of neuroleptics: The first in vivo pharmacokinetics of trifluoperazine in the rat brain and the first in vivo spectrum of fluphenazine in the human brain. In: Biol Psychiatry 30, 1991, pp. 656-662. PMID 2386658 .
- ↑ JJH Ackerman et al .: Mapping of metabolites in whole animals by 31P NMR using surface coils. In: Nature 283, 1990, pp. 167-170. PMID 7350541 .
- ↑ JW Prichard et al .: Cerebral metabolic studies in vivo by 31P NMR. In: PNAS 80, 1983, pp. 2748-2751. PMID 6573678 .
- ↑ World Cup Pardridge: Blood-brain barrier drug targeting: the future of brain drug development. In: Mol Interv 3, 2003, pp. 90-105. PMID 14993430 (Review).
- ^ DJ Begley: Delivery of therapeutic agents to the central nervous system: the problems and the possibilities. In: Pharmacol Ther , 104, 2004, pp. 29-45. PMID 15500907 (Review).
- ↑ WM Pardridge: Why is the global CNS pharmaceutical market so under-penetrated? In: Drug Discovery Today , 7, 2002, pp. 5-7. PMID 11790589 .
- ^ AG de Boer, PJ Gaillard: Strategies to improve drug delivery across the blood-brain barrier. In: Clin Pharmacokinet , 46, 2007, pp. 553-576. PMID 17596102 (Review).
- ^ AG de Boer and PJ Gaillard: Drug targeting to the brain. In: Annu Rev Pharmacol Toxicol , 47, 2007, pp. 323-355. PMID 16961459 (Review).
- ↑ BT Hawkins, RD Egleton: Pathophysiology of the blood-brain barrier: animal models and methods. In: Curr Top Dev Biol 80, 2008, pp. 277-309. PMID 17950377 (Review).
- ^ A b c N. Weiss, F. Miller, S. Cazaubon, PO Couraud: The blood-brain barrier in brain homeostasis and neurological diseases. In: Biochim. Biophys. Acta 1788, 2009, pp. 842-857 PMID 19061857 (Review).
- ^ I. Elmas et al .: Effects of profound hypothermia on the blood-brain barrier permeability in acute and chronically ethanol treated rats. In: Forensic Science International 119, 2001, pp. 212-216. PMID 11376985 .
- ^ SC Phillips, BG Cragg: Weakening of the blood-brain barrier by alcohol-related stresses in the rat. In: J Neurol Sci , 54, 1982, pp. 271-278., PMID 7201507 .
- ↑ AK Singh et al .: Effects of chronic ethanol drinking on the blood brain barrier and ensuing neuronal toxicity in alcohol-preferring rats subjected to intraperitoneal LPS injection. In: Alcohol Alcohol , 42, 2007, pp. 385-399. PMID 17341516 .
- ↑ a b J. Haorah et al .: Alcohol-induced blood-brain barrier dysfunction is mediated via inositol 1,4,5-triphosphate receptor (IP3R) -gated intracellular calcium release. In: J Neurochem , 100, 2007, pp. 324-336, PMID 17241155 .
- ↑ J. Haorah et al .: Ethanol-induced activation of myosin light chain kinase leads to dysfunction of tight junctions and blood-brain barrier compromise. Alcoholism. In: Clinical and Experimental Research 29, 2005, pp. 999-1009. PMID 15976526 .
- ↑ J. Haorah et al .: Alcohol-induced oxidative stress in brain endothelial cells causes bloodbrain barrier dysfunction. ( Memento of March 16, 2010 in the Internet Archive ) In: Journal of Leukocyte Biology , 78, 2005, pp. 1223-1232. PMID 16204625 .
- ^ R. Peters et al .: Smoking, dementia and cognitive decline in the elderly, a systematic review. In: BMC Geriatr 8, 2008, p. 36. PMID 19105840 (Review).
- ↑ a b P. R. Lockman et al .: Brain uptake kinetics of nicotine and cotinine after chronic nicotine exposure. In: J Pharmacol Exp Ther 314, 2005, pp. 636-642. PMID 15845856 .
- ↑ TJ Abbruscato et al .: Nicotine and cotinine modulate cerebral microvascular permeability and protein expression of ZO-1 through nicotinic acetylcholine receptors expressed on brain endothelial cells. In: J Pharm Sci 91, 2002, pp. 2525-2538. PMID 12434396 .
- ↑ BT Hawkins et al .: Nicotine increases in vivo blood-brain barrier permeability and alters cerebral microvascular tight junction protein distribution. In: Brain Res 1027, 2004, pp. 48-58. PMID 15494156 .
- ↑ YH Chen et al .: Enhanced Escherichia coli invasion of human brain microvascular endothelial cells is associated with alternations in cytoskeleton induced by nicotine. In: Cell Microbiol 4, 2002, pp. 503-514. PMID 12174085 .
- ↑ PR Lockman et al .: Chronic nicotine exposure alters blood-brain barrier permeability and diminishes brain uptake of methyllycaconitine. In: J Neurochem 94, 2005, pp. 37-44. PMID 15953347 .
- ↑ M. Kotlyar and DK Hatsukami: Managing nicotine addiction. In: J Dent Educ 66, 2002, pp. 1061-1073. PMID 12374267 .
- ^ PR Pentel et al .: A nicotine conjugate vaccine reduces nicotine distribution to brain and attenuates its behavioral and cardiovascular effects in rats. In: Pharmacol Biochem Behav 65, 2000, pp. 191-198. PMID 10638653 .
- ^ DE Keyler et al .: Maternal vaccination against nicotine reduces nicotine distribution to fetal brain in rats. In: J Pharmacol Exp Ther 305, 2003, pp. 587-592. PMID 12606612 .
- ↑ MG LeSage et al .: Effects of a nicotine conjugate vaccine on the acquisition and maintenance of nicotine self-administration in rats. In: Psychopharmacology 184, 2006, pp. 409-416. PMID 15991003 .
- ^ DA D'Andrea et al .: Microwave effects on the nervous system. In: Bioelectromagnetics 6, 2003, pp. 107-147. PMID 14628310 (Review).
- ↑ TH Patel et al .: Blood brain barrier (BBB) dysfunction associated with increased expression of tissue and urokinase plasminogen activators following peripheral thermal injury. In: Neurosci Lett 444, 2008, pp. 222-226. PMID 18718505 .
- ^ I. Ruppe: Structure and function of the blood-brain barrier. ( Memento of February 16, 2010 in the Internet Archive ) (PDF; 113 kB) In: Newsletter 1, 2003, pp. 15–17.
- ^ BR Persson et al .: Increased permeability of the blood-brain barrier induced by magnetic and electromagnetic fields. In: Ann NY Acad Sci 649, 1992, pp. 356-358. PMID 1580510 .
- ^ LG Salford et al .: Nerve cell damage in mammalian brain after exposure to microwaves from GSM mobile phones. In: Environ Health Perspect 111, 2003, pp. 881-883. PMID 12782486 .
- ↑ H. et al .: Nittby radio frequency and extremely low-frequency electromagnetic field effects on the blood-brain barrier. In: Electromagn Biol Med 27, 2008, pp. 103-126. PMID 18568929 (Review).
- ↑ JL Eberhardt et al .: Blood-brain barrier permeability and nerve cell damage in rat brain 14 and 28 days after exposure to microwaves from GSM mobile phones. In: Electromagn Biol Med 27, 2008, pp. 215-229. PMID 18821198 .
- ^ LG Salford et al .: Permeability of the blood-brain barrier induced by 915 MHz electromagnetic radiation, continuous wave and modulated at 8, 16, 50, and 200 Hz. In: Microsc Res Tech 27, 1994, pp. 535-542 . PMID 8012056 .
- ↑ A. Schirmacher et al .: Electromagnetic fields (1.8 GHz) increase the permeability to sucrose of the blood-brain barrier in vitro. In: Bioelectromagnetics 21, 2000, pp. 338-345. PMID 10899769 .
- ↑ H. Franke et al .: Electromagnetic fields (GSM 1800) do not alter blood-brain barrier permeability to sucrose in models in vitro with high barrier tightness. In: Bioelectromagnetics 26, 2005, pp. 529-535. PMID 16142784 .
- ^ HJ Weinmann et al .: Characteristics of Gadolinium-DTPA Complex: A Potential NMR Contrast Agent. In: Am J Roentgenol 142, 1984, pp. 619-624. PMID 6607655 .
- ↑ RC Brasch et al .: Contrast-enhanced NMR imaging: animal studies using gadolinium-DTPA complex. In: Am J Roentgenol 142, 1984, pp. 625-630. PMID 6607656 .
- ↑ VM Runge et al .: Visualization of Blood-Brain Barrier Disruption on MR Images of Cats with Acute Cerebral Infarction: Value of Administering a High Dose of Contrast Material. In: AJR Am J Roentgenol 162, 1994, pp. 431-435. PMID 8310940 .
- ↑ MA Ibrahim et al .: Magnetic resonance imaging relaxation times and gadolinium-DTPA relaxivity values in human cerebrospinal fluid. In: Invest Radiol 33, 1998, pp. 153-162. PMID 9525754 .
- ↑ AV Alexandrov et al .: Clinical significance of increased uptake of HMPAO on brain SPECT scans in acute stroke. In: J Neuroimaging , 6, 1996, pp. 150-155. PMID 8704289 (review).
- ↑ JC Masdeu, J. Arbizu: Brain single photon emission computed tomography: technological aspects and clinical applications. In: Semin Neurol 28, 2008, pp. 423-434. PMID 18843571 (Review).
- ↑ M. Essig: CT imaging diagnostics for a stroke. In: Visions 12, 2005, pp. 15-17.
- ^ KA Miles: Perfusion CT for the assessment of tumor vascularity: which protocol? In: Br J Radiol 76, 2003, pp. 36-42. PMID 15456712 .
- ↑ DA Leggett et al .: Blood-brain barrier and blood volume imaging of cerebral glioma using functional CT: a pictorial review. In: Eur J Radiol 30, 1999, pp 185-190. PMID 10452716 (Review).
- ↑ P. Ehrlich: The organism's need for oxygen: A color-analytical study. August Hirschwald, Berlin, 1885, 167 p. (Paul Ehrlich's habilitation thesis).
- ↑ P. Ehrlich: About the relationship between chemical constitution, distribution and pharmacological effect. Collected work on immunity research. August Hirschwald, Berlin, 1904, p. 574.
- ↑ EE Goldmann: The external and internal secretion of the healthy and diseased organism in the light of the vital color. In: Beitr Klin Chirurg 64, 1909, pp. 192-265.
- ↑ EE Goldmann: vital staining on the central nervous system. In: Abh. K. Preuss. Akad. Wiss. Phys. Med. 1, 1913, pp. 1-60.
- ↑ A. Biedl, R. Kraus: About a previously unknown toxic effect of bile acids on the central nervous system. In: Zentralblatt Innere Medizin , 19, 1898, pp. 1185–1200.
- ^ M. Lewandowsky: On the doctrine of the cerebrospinal fluid. In: Zentralblatt Klinische Medizin , 40, 1900, pp. 480–494.
- ^ CS Roy, CS Sherrington: On the regulation of the blood supply of the brain. (PDF; 3.7 MB) In: J Physiol , 11, 1890, pp. 85-108.
- ↑ AA Vein: Lina Stern: Science and fate .
- ↑ FK Walter: The general principles of the exchange of substances between the central nervous system and the rest of the body. In: Arch Psychiatr Nervenkr 101, 1930, pp. 195-230.
- ↑ H. Spatz: The importance of the vital color for the theory of the exchange of substances between the central nervous system and the rest of the body. In: Arch Psychiatr Nervenkr 101, 1933, pp. 267-358.
- ^ U. Friedemann: Blood-brain barrier. In: Physiol Rev 22, 1942, pp. 125-145.
- ↑ RD Tschirgi: Blood-brain barrier: fact or fancy? In: Fed Proc 21, 1962, pp. 665-671. PMID 13922779 .
- ^ G. Miller: Drug targeting. Breaking down barriers. In: Science 297, 2002, pp. 1116-1118. PMID 12183610 .
- ↑ TS Reese, MJ Karnovsky: Fine structural localization of a blood-brain barrier to exogenous peroxidase. In: J Cell Biol 34, 1967, pp. 207-217. PMID 6033532 .