Photorespiration
Parent |
Recovery process |
Gene Ontology |
---|
QuickGO |
The photorespiration ( Greek φῶς Phos , light; lat. : Respiratio , respiration ), also oxidative photosynthetic carbon cycle or (oxidative) C 2 cycle is a pathway in organisms that an oxygenic photosynthesis operate ( plants , algae , cyanobacteria ). Here, carbon dioxide is released in a light-dependent reaction and oxygen is consumed as in breathing. This is why the metabolic pathway is also called “light breathing”. This term is based on that of cellular respiration ("dark respiration"), since this is where carbon dioxide is also produced and oxygen is consumed. However, both processes have nothing to do with each other.
Photorespiration can occur in the course of carbon dioxide fixation in the Calvin cycle during photosynthesis. The key enzyme involved, RuBisCO, normally uses carbon dioxide as a substrate , but it also accepts oxygen as an alternative . This creates the toxic metabolic product 2-phosphoglycolate, which can no longer be used in the Calvin cycle and therefore has to be converted by other biochemical reactions. In higher plants these reactions take place in three closely spaced cell compartments : the chloroplasts , the peroxisomes, and the mitochondria . It is essentially a recovery process .
The photorespiratory pathway is considered to be one of the most wasteful processes on earth.
Discovery story

The first records of the effects of photorespiration come from Otto Warburg . In 1920 he observed in the freshwater alga Chlorella that the absorption of carbon dioxide can be inhibited by oxygen. The scientist John P. Decker was able to prove that in the presence of light and oxygen, more carbon dioxide is released. Without knowing the biochemical processes, the two scientists were able to draw conclusions about the importance of oxygen in photorespiration.
Further steps in the Enlightenment dealt with the role of glycolate as one of the first metabolic products of photorespiration and dealt with the influence of oxygen and carbon dioxide on this process.
In 1960 Warburg and Günter Krippahl were able to show that high oxygen concentrations cause the formation of glycolate. This was confirmed two years later by James A. Bassham and Martha Kirk in Chlorella , who were also able to measure an oxygen-dependent increase in glycolate and 2-phosphoglycolate, respectively. Israel Zelitch hypothesized in 1964 that glycolate was an important intermediate in photorespiration. In addition, Bassham and Kirk observed that oxygen can inhibit photosynthesis.
The formation of glycolate caused by oxygen is reversed by high concentrations of carbon dioxide, as was demonstrated by Bermingham and co-workers the following year. This shows that oxygen and carbon dioxide compete with each other. In 1966, these results were validated by Gleb Krotkov's working group: the oxygen-dependent inhibition of photosynthesis is reduced by increasing CO 2 concentrations.
The first theory about the photorespiratory pathway was proposed in 1971 by Nathan E. Tolbert .
Based on the previous observations and their own investigations, George E. Bowes and William L. Ogren were able to demonstrate in the same year with the enzyme RuBisCO ( ribulose 1,5-bisphosphate carboxylase / oxygenase ) isolated from soybeans that both carbon dioxide and oxygen are substrates for RuBisCO serve. At this point in time, RuBisCO was still called “ribulose bisphosphate carboxylase ”, since only its carboxylating , i.e. carbon- assimilating , function was known. If RuBisCO reacts with oxygen instead of carbon dioxide, 2-phosphoglycolate is formed. The enzyme thus has both a carboxylase and an oxygenase function , which is how the name used today comes from. The abbreviation RuBisCO for the enzyme was introduced by David Eisenberg at a seminar in 1979.
These conclusions were initially accepted only hesitantly. In 1973, however, Tolbert's group was able to provide unequivocal evidence of the oxygenase reaction of RuBisCO with isotope-labeled substrates ( 14 C-ribulose-1,5-bisphosphate and 18 O 2 ). Through these results and the previous work and theories, Tolbert is listed in the literature as the discoverer of photorespiration.
Further investigations in the following years dealt with the measurements of the reaction kinetics of RuBisCO, the influence of temperature on photorespiration and the characterization of all enzymes involved. Knowledge of the translocators for the exchange of metabolites between the organelles, however, is still limited and remains the subject of research.
Biochemistry of the oxygenase function

Green plants, algae and cyanobacteria ("blue-green algae") absorb carbon dioxide in order to build up carbohydrates from it in the Calvin cycle. The first step is carried out by the enzyme ribulose-1,5-bisphosphate-carboxylase / -oxygenase (RuBisCO), which catalyzes the addition of CO 2 to ribulose-1,5-bisphosphate ( 1 , see figure top branch). As a result, two molecules of 3-phosphoglycerate ( 3 , upper figure) are formed and processed further in the Calvin cycle.
As a side reaction, RuBisCO also accepts oxygen (“oxygenase reaction”), which creates 2-phosphoglycolate ( 4 ) in addition to 3-phosphoglycerate ( 3 ) (see figure on the lower branch). 3-phosphoglycerate is a regular part of the Calvin cycle. However, 2-phosphoglycolate cannot be directly converted into a carbohydrate, nor is it required for metabolism in any form. Green algae, for example, excrete its dephosphorylated form, glycolate, when there is a good supply of CO 2 (photosynthetic glycolate excretion) .
Photorespiration is a metabolic pathway that converts 2-phosphoglycolate into 3-phosphoglycerate through a series of reactions and thus counteracts carbon loss. Nine enzymes are required for this regeneration; in higher plants it takes place with the participation of the cytosol in the chloroplast, in the peroxisome and in the mitochondrion.
The formation of 2-phosphoglycolate in the chloroplast takes place through RuBisCO exclusively in the light, since the enzyme is not active in the dark. This explains the first part in the name Photo respiration. This means that photorespiration always runs parallel to the Calvin cycle.
Cause and temperature dependence
The side reaction of the enzyme RuBisCO and the associated occurrence of the photorespiratory metabolic pathway is due to the fact that RuBisCO converts both CO 2 and O 2 as a substrate. Although the affinity of RuBisCO for CO 2 is higher than for O 2 , the K M value for CO 2 is 9 μmol / l, for O 2 350 μmol / l and thus CO 2 is preferred over O 2 , but the concentration is preferred of oxygen in water 20 times higher than that of CO 2 . As a result, every fourth to every second molecule of ribulose-1,5-bisphosphate is reacted with oxygen instead of carbon dioxide.
temperature | CO 2 concentration in the water in µM | O 2 concentration in the water in µM | Concentration ratio CO 2 / O 2 |
---|---|---|---|
5 ° C | 21.93 | 401.2 | 0.0515 |
15 ° C | 15.69 | 319.8 | 0.0462 |
25 ° C | 11.68 | 264.6 | 0.0416 |
35 ° C | 9.11 | 228.2 | 0.0376 |
Since RuBisCO can use both carbon dioxide and oxygen as a substrate, the oxygenase reaction of RuBisCO occurs more frequently the higher the oxygen concentration is relative to the carbon dioxide concentration. This ratio increases with temperature. The solubility of a gas falls with increasing temperature, but more with CO 2 than with O 2 (see table). With the same ratio of the partial pressures of the two gases, the ratio of dissolved CO 2 to dissolved O 2 therefore decreases with increasing temperature. It is therefore less favorable for CO 2 fixation. In addition, the stomata of the leaf are closed at higher temperatures in order to reduce the loss of water by the plant. This means that less CO 2 also gets into the cell, while the local O 2 content increases due to photolysis . As a result, high temperatures favor photorespiration.
Process of photorespiration
The Glycolate Path
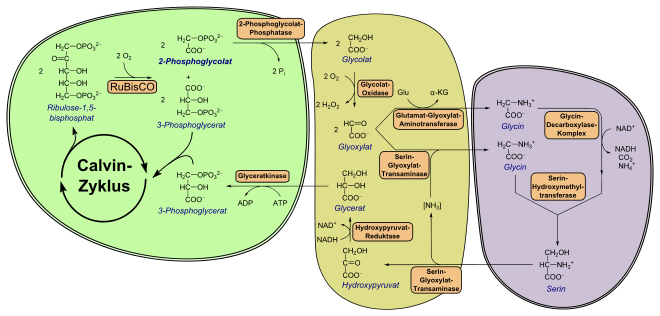
The 2-phosphoglycolate formed in the chloroplast is first converted to glycolate by a 2-phosphoglycolate phosphatase (PGP, EC 3.1.3.18 ). The enzyme activity is essential for plants, if the enzyme is missing, for example, they can not grow under natural CO 2 concentrations. Although plants also have a cytosolic PGP, only the plastid isoenzyme plays a role in photorespiration.
Glycolate is transported out of the chloroplast by a glycolate-glycerate antiporter and enters the peroxisome through porin- like channels. In assimilating leaf cells of higher plants there is a special type of peroxisome, which is why it is called a leaf peroxisome .
In the peroxisome, glycolate is oxidized to glyoxylate by a flavin mononucleotide (FMN) -dependent glycolate oxidase ( EC 1.1.3.15 ) . The enzyme exists as a tetra- or octamer of identical subunits. While corn has only one gene copy of this enzyme, were in the thale cress five copies identified. If these gene copies are removed, these plants can only grow under high CO 2 concentrations. Oxygen is consumed during the oxidation to glyoxylate, which refers to the second part of the term photo respiration : As is generally the case in aerobic respiration, oxygen is consumed and later carbon dioxide is released (see below).
This step produces hydrogen peroxide (H 2 O 2 ), which is toxic to the cell. Therefore, H 2 O 2 is broken down into water and oxygen (O 2 ) by a catalase .

The glyoxylate is converted by two enzymes:
- as α-keto acid is glyoxylate by the serine-glyoxylate transaminase ( EC 2.6.1.45 ), a homodimer to glycine transaminated ; the donor of the NH 2 group is the L - serine that is only formed subsequently . The enzyme prefers L- serine as nitrogen donor ; it is essential for the photorespiratory pathway.
- Another molecule of glyoxylate is converted to glycine by a glutamate glyoxylate aminotransferase ( EC 2.6.1.4 ), with L - glutamate (Glu) serving as the amino group donor . This creates α-ketoglutarate . In addition to L -glutamate, this aminotransferase can also use L -alanine as an N-donor.
The two glycine molecules produced are ultimately transported into the mitochondrion. There they combine in a tetrahydrofolic acid (THF) -dependent reaction to form a molecule of L- serine. Both a glycine decarboxylase complex (GDC) and a serine hydroxymethyltransferase (SHMT, EC 2.1.2.1 ) are involved (see figure above). Functionally, GDC consists of three enzymes, a decarboxylating glycine dehydrogenase ( EC 1.4.4.2 ), an aminomethyltransferase ( EC 2.1.2.10 ) and a dihydrolipoyl dehydrogenase ( EC 1.8.1.4 ). GDC deaminates and decarboxylates glycine, consuming NAD + . The CO 2 produced during this step may come out of the mitochondrion in the form of bicarbonate . In C 3 plants, 30–50% of the released carbon dioxide is re-fixed by RuBisCO, the rest escapes. SHMT finally links the methylene group of the first glycine, water and another molecule of glycine to form L -serine.
Both enzyme complexes, GDC and SHMT, are highly concentrated in the matrix of plant cell mitochondria and are sensitive to oxidation. The released ammonium (NH 4 + ) is not lost and is still regenerated in the photorespiratory nitrogen cycle (see section below).
After transport into the peroxisome, L -serine is deaminated to hydroxypyruvate by the serine glyoxylate transaminase described above. This is reduced to D- glycerate with consumption of NADH , which catalyzes an NAD + -dependent hydroxypyruvate reductase ( EC 1.1.1.81 ). Back in the chloroplast, D- glycerate is converted to 3-phosphoglycerate by a glycerate kinase (GKK, EC 2.7.1.31 ), in which one molecule of ATP is invested. 3-phosphoglycerate then enters the Calvin cycle regularly. Interestingly, the GKK is the only known enzyme that forms 3-phosphoglycerate; Bacteria, on the other hand, use a kinase that produces 2 -phosphoglycerate.
The metabolites involved are exchanged either by translocators or by porins (in peroxisomes).
Regeneration of glutamate (photorespiratory nitrogen cycle)
In the photorespiratory pathway, glutamate is converted to α-ketoglutarate in the peroxisome. The amino group is later split off in the mitochondrion by the glycine decarboxylase complex in the form of NH 4 + ( ammonium ). Ammonium itself has a cytotoxic effect in higher concentrations, but must not simply be excreted. Because plant growth is often limited by the availability of nitrogen.
So that ammonium as a valuable nitrogen source is not lost and glutamate is regenerated, further reactions in the chloroplast follow. NH 4 + gets there by an ammonium transporter, possibly also by simple diffusion. L -glutamate (3, see figure) and NH 4 + are then converted into L - glutamine (2) by the glutamine synthetase (GS, EC 6.3.1.2 ) while consuming ATP . The latter is converted with α-ketoglutarate (1) by a ferredoxin- dependent glutamine-oxoglutarate aminotransferase (GOGAT, also glutamate synthase, EC 1.4.7.1 ) to form two molecules of L- glutamate. At the same time, two molecules of ferredoxin are oxidized (Fd ox ). In exchange for malate, α-ketoglutarate enters the chloroplast (DiT1 translocator), while glutamate is transported back into the peroxisome by a malate-glutamate translocator (DiT2). There it is available again for the transamination of glyoxylate.
Almost all released ammonium is refixed by this cycle, only about 0.1 ‰ is lost. This regeneration consumes a total of two molecules of ferredoxin and one molecule of ATP:
Metabolism in Cyanobacteria
Cyanobacteria (blue-green algae) are the only known bacteria that carry out oxygenic photosynthesis. They use the Calvin cycle to fix carbon dioxide. For a long time it was assumed that photorespiration does not occur in cyanobacteria for two reasons. Firstly, cyanobacteria actively enrich carbon dioxide through carboxysomes, so that RuBisCO hardly reacts with oxygen and therefore photorespiration does not occur to any significant extent anyway. Second, it was believed that if small amounts of glycolate were to form, it would be excreted like in green algae and need not be regenerated in any form.
It is now known that cyanobacteria also have a 2-phosphoglycolate metabolic pathway. Similar to plants, this begins with the conversion of 2-phosphoglycolate via glycolate to glyoxylate. For the second step, the filamentous, nitrogen-fixing cyanobacterium Anabaena and the marine Prochlorococcus marinus use a plant-like glycolate oxidase to oxidize glycolate to glyoxylate. Synechocystis , on the other hand, uses a glycolate dehydrogenase that consumes NADH and does not produce hydrogen peroxide.
Glyoxylate can - depending on the type of cyanobacterium - be metabolized differently; three metabolic pathways, some of which overlap, were identified. In a few cyanobacteria it is oxidized to oxalate by a glyoxylate oxidase with consumption of oxygen . Oxalate is then converted into two molecules of carbon dioxide by an oxalate decarboxylase and a format dehydrogenase, and one molecule of NADH is also formed (decarboxylation pathway) . This path does not serve to recirculate carbon, on the contrary, it is released as a result.
Other cyanobacteria produce hydroxypyruvate from glyoxylate. This takes place either via a plant-like mechanism ( plant-like metabolic pathway , see section above). Alternatively, some cyanobacteria can convert two molecules of glyoxylate into hydroxypyruvate by means of a glyoxylate carboligase and a tartronic acid semialdehyde reductase with consumption of NADH, which also produces carbon dioxide and tartronate semialdehyde as an intermediate product (glycerate pathway ) .
In Synechocystis and Anabaena , hydroxypyruvate - as in plants - is phosphorylated by a plant-like glycerate kinase to 3-phosphoglycerate with consumption of ATP. However, other cyanobacteria first form 2-phosphoglycerate , which is then isomerized to 3-phosphoglycerate.
In Synechocystis , these three overlapping metabolic pathways even occur together. Synechocystis only needs high carbon dioxide concentrations to survive if all three pathways are interrupted - analogous to that in plants in which the photorespiratory pathway is interrupted.
Biological avoidance
Photorespiration is an expensive process in which more ATP and reducing agents are invested. Without photorespiration, 3 mol of ATP and 2 mol of NADPH would be metabolized for each fixed mol of CO 2 . In the event that the ratio of carboxylation to oxygenation is 1 to 0.25, the consumption per fixed mol of CO 2 increases to 5.375 mol of ATP and 3.5 mol of NADPH.
This additional consumption reduces the efficiency of photosynthesis. Only under sufficiently high CO 2 partial pressures (e.g. 1% CO 2 ) will there be no oxygenase reaction and therefore no loss of efficiency in photosynthesis. Thus, photorespiration per se represents an energetically unfavorable additional investment for the C 3 plant. It is estimated that the carbon gain in the Calvin cycle without photorespiration could be about 30% higher. Because RuBisCO is the most abundant protein on earth, photorespiration is even classified as one of the most wasteful processes on earth.
In the course of evolution , various mechanisms have developed to avoid the costly side reaction of RuBisCO, especially with regard to decreased CO 2 concentrations. The green algae and cyanobacteria living in the water have carbon dioxide-concentrating mechanisms such as pyrenoids or carboxysomes . These have the function of enriching carbon dioxide around the RuBisCO molecule. This creates a high local CO 2 concentration at which RuBisCO hardly reacts with oxygen.
In the land plants emerged due to changed climate conditions C 4 - and CAM metabolism . These are based on an ATP-driven CO 2 pump with which they actively increase the CO 2 concentration in the tissue and thus saturate the RuBisCO with carbon dioxide. When the temperature rises, they hardly suffer any loss of photosynthetic efficiency, since photorespiration occurs only to a small extent. As a result, they have a higher net fixation rate than C 3 plants. C 4 plants include, for example, sugar cane , sorghum , maize and many weeds that can be found in hot locations.
meaning
Thanks to the photorespiratory pathway, all organisms that carry out oxygenic photosynthesis (plants, algae, cyanobacteria) have a metabolic pathway to keep the carbon loss as a result of the oxygenase reaction of RuBisCO as low as possible.
In the multi-stage process, three carbon atoms are made available from two molecules of 2-phosphoglycolate for the Calvin cycle and one molecule of CO 2 is released. If this CO 2 molecule is not refixed in the Calvin cycle, the loss of carbon atoms through photorespiration is 25%.
Thus the primary function of photorespiration is to recover the carbon. In most green C 3 plants, including C 4 plants such as maize , and in cyanobacteria, the metabolic pathway is even essential, i.e. indispensable.
C 4 plants and cyanobacteria actively enrich carbon dioxide, so that photorespiration does not occur as strongly as in C 3 plants. Nevertheless, one can also observe the photorespiratory path there. This means that photorespiration has not disappeared in the course of evolution, despite carbon-dioxide-concentrating mechanisms, and must therefore offer some other advantages:
- High concentrations of 2-phosphoglycolate, glyoxylate or glycine have a metabolically toxic effect. For example, 2-phosphoglycoate inhibits the triose phosphate isomerases and glyoxylate inhibits RuBisCO. Land plants cannot excrete these metabolites like green algae. However, due to photorespiration, the concentrations remain below harmful limit values.
- During photorespiration amino acids such as glycine and L- serine are formed, in higher plants it is the main source of these amino acids. However, these amino acids could also be synthesized by other metabolic pathways, especially if photorespiration is suppressed.
- In field cress and in common wheat it was shown that when photorespiration was suppressed (2% atmospheric oxygen concentration), nitrate assimilation was also inhibited.
- One advantage that is often discussed in the literature is the ability to cope with certain stressful situations. With high light intensities, low or high temperatures and in particular a lack of water, photo-oxidative damage to the photosynthetic apparatus increases. This is because the photosynthetic reaction centers are overloaded because the electrons provided cannot be used up quickly enough in the Calvin cycle (in the “dark reaction”) - the electron transport “stalls”. Due to the increased consumption of reductants during photorespiration, especially under low CO 2 partial pressures, this metabolic pathway could be interpreted as a kind of "protective valve". The decarboxylation path used by a few cyanobacteria is used exclusively to remove excess energy, since 2-phosphoglycerate is not recycled. For land plants, however, it must be noted that the majority of this excess energy (50–70% of all absorbed photons) is eliminated in the so-called xanthophyll cycle .
- One possibility that has recently been discussed is that the hydrogen peroxide (H 2 O 2 ) generated during photorespiration is important for signaling processes. H 2 O 2 is considered to be an important signaling molecule because it influences other redox signals, among other things. In this way, for example, processes of plant growth and stress responses (pest infestation) are controlled. Since H 2 O 2 is formed fastest in photosynthetically active cells through photorespiration, the molecule could, for example, activate the plant's defense system.
evolution
organism | |
---|---|
Rhodopseudomonas sphaeroides (bacteria) | 9 |
Cyanobacteria (blue-green algae) | 50 |
Euglena ( Euglenozoa ) | 54 |
Chlorococcales ( green algae ) | 62 |
C 3 - angiosperms | 80 |
C 4 angiosperms | 64-80 |
The precursors of today's cyanobacteria were the first living beings with oxygenic photosynthesis. This meant that RuBisCO was also exposed to oxygen. 2-phosphoglycolate was probably also produced there, as the CO 2 -concentrating mechanisms, such as e.g. B. the carboxysome, emerged much later in evolution (probably 360-300 million years ago, when the oxygen concentration in the atmosphere increased). Over time, plants have lost enzymes for the glycerate pathway, which is still found in many cyanobacteria today. The photorespiration has been preserved. Even in picoplankton , the genome of which is greatly reduced (e.g. Prochlorococcus or Synechococcus ), the genes for photorespiration have been preserved. The C 2 cycle of today's cyanobacteria was either already present at the beginning or developed quite early in the first protocyanobacteria.
According to the endosymbiont theory , the chloroplasts of today's plants and algae can be traced back to the precursors of the cyanobacteria, so that RuBisCO also got into these organisms. RuBisCO of all organisms (bacteria, algae, plants) has in common that they accept both carbon dioxide and oxygen as a substrate. A specificity constant can be used to indicate how much RuBisCO prefers CO 2 as a substrate over O 2 . This constant is the product of two ratios: the Michaelis constant K M and the maximum rate constant v max (see table).
In the course of evolution, the affinity of the RuBisCO for CO 2 was only slightly improved. The catalytic center of the enzyme was probably optimized during the time when the atmospheric O 2 concentration was still very low and thus could not exert selective pressure. As a result, RuBisCO could no longer be significantly improved by the later increase in the oxygen concentration.
Influence on world nutrition
In C 3 plants, photorespiration occurs particularly under warm and dry environmental conditions, which reduces crop yields in those regions - especially with regard to global warming and the growing world population . Part of the research is therefore oriented towards a genetic reduction in photorespiration or the introduction of new metabolic pathways, which could increase crop yields. By breaking down the 2-phosphoglycolate directly in the chloroplast, the efficiency of light utilization in tobacco plants could be increased by 17% and crop yields increased by more than 40%.
Various attempts to increase the specificity of RuBisCO for carbon dioxide resulted in lower conversion rates. This worsened the rate of photosynthesis. Another strategy pursues the goal of introducing other RuBisCO species, such as the bacterial type II RuBisCO, into C 3 plants. However, this failed because the newly introduced RuBisCO does not form a functional enzyme.
Another part of the research therefore tries not to suppress photorespiration in C 3 plants. Instead, it pursues the goal of converting C 3 plants into C 4 plants, since photorespiration hardly occurs there and C 4 plants take advantage of C 3 plants when there is a lack of water and nitrogen , especially when temperatures rise. One of these projects is the so-called C 4 rice, in which C 4 photosynthesis is to be introduced into commercially available rice, a C 3 plant .
literature
- Bauwe H., Hagemann M. and Fernie AR. (2010): Photorespiration: players, partners and origin . In: Trends Plant Sci . 15 (6); 330-336; PMID 20403720 ; doi : 10.1016 / j.tplants.2010.03.006
- Reumann, S. and Weber, AP. (2006): Plant peroxisomes respire in the light: some gaps of the photo respiratory C 2 cycle have become filled - others remain . In: Biochim Biophys Acta 1763 (12); 1496-1510; PMID 17046077 ; doi : 10.1016 / j.bbamcr.2006.09.008
- Foyer, CH. et al . (2009): Photorespiratory metabolism: genes, mutants, energetics, and redox signaling . In: Annu Rev Plant Biol . 60; 455-484; PMID 19575589 ; doi : 10.1146 / annurev.arplant.043008.091948
- Bauwe, H. (2010): Recent developments in photorespiration research . In: Biochem Soc Trans . 38 (2); 677-682; PMID 20298242 ; doi : 10.1042 / BST0380677
- Hans W. Heldt, Birgit Piechulla: Plant biochemistry . 4th edition. Spectrum Academic Publishing House, Heidelberg 2008; ISBN 978-3-8274-1961-3
- Caroline Bowsher, Martin W. Steer, Alyson K. Tobin: Plant Biochemistry . Garland Pub, New York, NY 2008, ISBN 978-0-8153-4121-5
- Nelson, David L., Cox, Michael M., Lehninger, Albert L. [Ent.]: Lehninger Biochemie. Springer, Berlin; 4th, completely revised u. exp. Edition 2009; ISBN 978-3-540-68637-8
- Andreas Bresinsky , Christian Körner, Joachim W. Kadereit, G. Neuhaus, Uwe Sonnewald: Strasburger - textbook of botany . 36th edition. Spectrum Akademischer Verlag, Heidelberg 2008, ISBN 978-3-8274-1455-7
- Ulrich Lüttge , Manfred Kluge, Gabriela Bauer: Botany . 5. completely revised Edition. Wiley-VCH, Weinheim 2005; ISBN 978-3-527-31179-8
Individual evidence
- ^ A b c Andreas Bresinsky, Christian Körner, Joachim W. Kadereit, G. Neuhaus, Uwe Sonnewald: Strasburger - Textbook of Botany . 36th edition. Spektrum Akademischer Verlag, Heidelberg 2008, ISBN 978-3-8274-1455-7 ; P. 304 ff.
- ↑ a b foyer, CH. et al . (2009): Photorespiratory metabolism: genes, mutants, energetics, and redox signaling . In: Annu Rev Plant Biol. 60 ; 455-484; PMID 19575589 ; doi : 10.1146 / annurev.arplant.043008.091948 .
- ^ Warburg, O. (1920): About the rate of photochemical carbonic acid decomposition in living cells. II. In: Biochem. Line 103; 188-217.
- ^ Decker, JP. (1955): A rapid, postillumination deceleration of respiration in green leaves . In: Plant Physiol . 30 (1); 82-84; PMID 16654735 ; PMC 540603 (free full text).
- ↑ Warburg, O. and Krippahl, G .: glycolic acid formation in chlorella. In: Journal of Nature Research B . 15, 1960, pp. 197-199 ( PDF , free full text). .
- ^ Bassham, YES. and Kirk, M. (1962): The effect of oxygen on the reduction of CO2 to glycolic acid and other products during photosynthesis by Chlorella. In: Biochem Biophys Res Commun . 9 (5); 376-380; PMID 13969890 ; doi : 10.1016 / 0006-291X (62) 90019-0 .
- ↑ Zelitch, I. (1964): Organic acids and respiration in photosynthetic tissues . In: Ann. Rev. Plant Physiol . 15; 121-142; doi : 10.1146 / annurev.pp.15.060164.001005 .
- ^ Whittingham, CP, Hiller, RG. and Bermingham, M. (1963): The production of glycollate during photosynthesis . In: Photosynthesis mechanisms in green plants. B. Kok and AT. Jagendorf, pp. 675-683. Washington: Natl. Acad. Sci.-Natl. Res. Counc. Publ. 1145.
- ↑ Forrester, ML., Krotkov, G. and Nelson, CD (1966): Effect of oxygen on photosynthesis, photorespiration and respiraion in detached leaves. I. Soybean . In: Plant Physiol. 41 (3); 422-427; PMID 16656271 ; PMC 1086359 (free full text).
- ↑ Tolbert, NE. (1971): Microbodies Peroxisomes and glyoxysomes . In: Ann. Rev. Plant Physiol . 22; 45-74; doi : 10.1146 / annurev.pp.22.060171.000401 .
- ↑ Ogren, WL. and Bowes, G. (1971): Ribulose diphosphate carboxylase regulates soybean photorespiration . In: Nature New Biol . 230 (13); 159-160; PMID 5279476 ; doi : 10.1038 / newbio230159a0
- ^ Bowes, G., Ogren, WL, Hageman, RH. (1971): Phosphoglycolate production catalyzed by ribulose diphosphate carboxylase . In: Biochem. Biophys. Res. Commun . 45; 716-722; PMID 4331471 ; doi : 10.1016 / 0006-291X (71) 90475-X .
- ^ Portis, AR. Jr. and Parry. MA. (2007): Discoveries in Rubisco (Ribulose 1,5-bisphosphate carboxylase / oxygenase): a historical perspective. In: Photosynth Res . 94 (1); 121-143; PMID 17665149 ; doi : 10.1007 / s11120-007-9225-6 .
- ↑ Andrews, TJ., Lorimer, GH. and Tolbert, NE. (1973): Ribulose diphosphate oxygenase. I. Synthesis of phosphoglycolate by fraction-1 protein of leaves. In: Biochemistry 12 (1); 11-18; PMID 4683476 ; doi : 10.1021 / bi00725a003 .
- ^ Lorimer, GH., Andrews, TJ. And Tolbert, NE. (1973): Ribulose diphosphate oxygenase. II. Further proof of reaction products and mechanism of action. In: Biochemistry 12 (1); 18-23; PMID 4683482 ; doi : 10.1021 / bi00725a004 .
- ↑ a b Peter Schopfer and Axel Brennicke: Plant Physiology . Elsevier, Munich 2006. ISBN 978-3-8274-1561-5 , pp. 227-231.
- ^ David Nelson, Michael Cox: Lehninger Biochemie . 4th, completely revised u. exp. Edition. Springer, Berlin 2009, ISBN 978-3-540-68637-8 , p. 787.
- ↑ a b c Reumann, S. and Weber, AP. (2006): Plant peroxisomes respire in the light: some gaps of the photo respiratory C 2 cycle have become filled - others remain . In: Biochim Biophys Acta 1763 (12); 1496-1510; PMID 17046077 ; doi : 10.1016 / j.bbamcr.2006.09.008
- ↑ Caroline Bowsher, Martin W. Steer, Alyson K. Tobin: Plant Biochemistry . Garland Pub, New York 2008, ISBN 978-0-8153-4121-5 , p. 118
- ↑ Peter H. Raven, Ray F. Evert, Susan E. Eichhorn: Biology of plants . 4th edition de Gruyter, Berlin, New York 2006, ISBN 978-3-11-018531-7 , pp. 149ff.
- ↑ a b c Hermann Bauwe: Photorespiration: The Bridge to C4 Photosynthesis . In: Agepati S. Raghavendra (Ed.) And Rowan F. Sage (Ed.): C4 Photosynthesis and Related CO2 Concentrated Mechanisms (Advances in Photosynthesis and Respiration). Springer Netherlands 2011; ISBN 978-90-481-9406-3 ; Pp. 81-108.
- ^ David Nelson, Michael Cox: Lehninger Biochemie . Springer, Berlin; 4th, completely revised u. exp. Edition 2009; ISBN 978-3-540-68637-8 ; P. 1042.
- ↑ D. Sültemeyer, T. Stuhlfauth, HP Fock: Is pseudocyclic ATP formation Involved in providing energy for the HCO3- -concentrating mechanism in blue green algae? In: Annual Meeting of the American Society of Pant Physiologists , St. Louis, Plant Physiology 4, Suppl., Page 161, abstract 967, PMC 1056509 (free full text).
- ↑ a b c d Bauwe H., Hagemann M. and Fernie AR. (2010): Photorespiration: players, partners and origin . In: Trends Plant Sci . 15 (6): 330-336; PMID 20403720 ; doi : 10.1016 / j.tplants.2010.03.006 .
- ^ Bauwe, H. (2010): Recent developments in photorespiration research . In: Biochem Soc Trans . 38 (2); 677-682; PMID 20298242 ; doi : 10.1042 / BST0380677 .
- ↑ a b Eisenhut, M. et al . (2008): The photorespiratory glycolate metabolism is essential for cyanobacteria and might have been conveyed endosymbiontically to plants . In: Proc Natl Acad Sci USA . Volume 105, number 44, November 2008, pp. 17199-17204, doi : 10.1073 / pnas.0807043105 , PMID 18957552 , PMC 2579401 (free full text).
- ↑ Maurino, VG. and Peter Hänsel, C. (2010): Photorespiration: current status and approaches for metabolic engineering . In: Curr Opin Plant Biol . 13 (3); 249-256; PMID 20185358 ; doi : 10.1016 / j.pbi.2010.01.006 .
- ^ Ulrich Lüttge, Manfred Kluge, Gabriela Bauer: Botany . 5. completely revised Edition. Wiley-VCH, Weinheim 2005, ISBN 978-3-527-31179-8 , p. 157.
- ↑ Elmar Weiler, Lutz Nover, Wilhelm Nultsch: General and molecular botany . Thieme Verlag, Stuttgart 2008, ISBN 978-3-13-147661-6 , p. 286.
- ↑ Zelitch, I. et al . (2009): High glycolate oxidase activity is required for survival of maize in normal air . In: Plant physiology. Volume 149, number 1, January 2009, pp. 195-204, doi : 10.1104 / pp.108.128439 , PMID 18805949 , PMC 2613714 (free full text).
- ↑ Anderson, LE. (1971): Chloroplast and cytoplasmic enzymes. II. Pea leaf triose phosphate isomerases. In: Biochim Biophys Acta 235 (1); 237-244; PMID 5089710 ; doi : 10.1016 / 0005-2744 (71) 90051-9
- ↑ Kleczkowski, LA. (1994): Inhibitors of Photosynthetic Enzymes / Carriers and Metabolism . In: Annual Review of Plant Physiology and Plant Molecular Biology 45; 339-367; doi : 10.1146 / annurev.pp.45.060194.002011 .
- ^ Ulrich Lüttge, Manfred Kluge, Gabriela Bauer: Botany . 5. completely revised Edition. Wiley-VCH, Weinheim 2005, ISBN 978-3-527-31179-8 , p. 158.
- ^ Peter hansel, C. et al. (2010): Photorespiration . In: Arabidopsis Book . 8th; e0130; PMID 22303256 ; doi : 10.1199 / tab.0130 .
- ↑ Caroline Bowsher, Martin W. Steer, Alyson K. Tobin: Plant Biochemistry . Garland Pub, New York, NY 2008, ISBN 978-0-8153-4121-5 , pp. 86ff.
- ↑ Hans W. Heldt , Birgit Piechulla: Plant biochemistry . 4th edition. Spektrum Akademischer Verlag, Heidelberg 2008, ISBN 978-3-8274-1961-3 , p. 110.
- ^ Ulrich Lüttge, Manfred Kluge, Gabriela Bauer: Botany . 5. completely revised Edition. Wiley-VCH, Weinheim 2005, ISBN 978-3-527-31179-8 , p. 139.
- ↑ Andersson, I. (2007): Catalysis and regulation in Rubisco . In: Journal of experimental botany. Volume 59, Number 7, 2008, pp. 1555-1568, doi : 10.1093 / jxb / ern091 , PMID 18417482 (review). PDF (free full text access)
- ↑ Peterhänsel, C. Niessen, M. and Kebeish, RM. (2008): Metabolic engineering towards the enhancement of photosynthesis . In: Photochem Photobiol . 84 (6); 1317-1323; PMID 18764897 ; doi : 10.1111 / j.1751-1097.2008.00427.x .
- ↑ Peterhänsel, C. and Maurino VG. (2010): Photorespiration redesigned . In: Plant physiology. Volume 155, number 1, January 2011, pp. 49-55, doi : 10.1104 / pp.110.165019 , PMID 20940347 , PMC 3075789 (free full text) (review).
- ↑ Donald R. Ort, Helen W. Liu, Amanda P. Cavanagh, Paul F. South: Synthetic glycolate metabolism pathways stimulate crop growth and productivity in the field . In: Science . tape 363 , no. 6422 , January 4, 2019, ISSN 1095-9203 , p. eaat9077 , doi : 10.1126 / science.aat9077 , PMID 30606819 ( sciencemag.org [accessed January 6, 2019]).
- ^ Homepage of the C 4 -Reis project .
Web links