Chlorophyll fluorescence
The chlorophyll fluorescence is a phenomenon of light absorption of chlorophyll . With the photochemical conversion of photons ( light quanta ) into chemical energy, as with all physical-chemical conversion processes, there is a power loss . In the case of chlorophyll, in addition to the desired performance (the transfer of electrons to the electron transport chain ), energy in the form of heat, phosphorescence or fluorescence is also given off.
In science one can make practical use of the property of chlorophyll fluorescence in order to measure photosynthetic activity non-destructively. This analysis can be used both to make statements about the condition of photosystem II and to examine the stress physiology of the plants. The method is also used in environmental protection. Here you can measure the degree of damage to forests, for example.
General
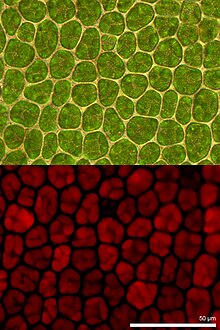
Basics
The absorption of visible light (wavelength range approx. 400–700 nanometers) is accompanied in photosynthetically active cells by the emission of weak light in the longer-wave range. This fluorescence is triggered by the emission of light energy by excited chlorophyll a molecules and follows the excitation of these molecules by irradiated light within nanoseconds. At room temperature, the chlorophylls of photosystem II, in particular of the reaction center P 680 and the associated antenna chlorophylls, are almost exclusively the triggers.
Pigment molecules are energetically stimulated by the absorption of light energy. In the case of chlorophyll, excitation in the blue light range generates the high-energy second singlet state , while excitation in the longer-wave (red light) range generates the first singlet state. The more energetic second singlet state can pass into the first singlet state with the release of thermal energy. The energy of the first singlet state can be used both for the energy transfer (exciton transfer) between the chlorophyll molecules of the antenna and in the reaction center P 680 for the photochemical electron transfer to the primary acceptor Q (“quencher”). However, the first singlet state can also revert to the ground state of the chlorophyll molecule due to fluorescence or heat emission. Alternatively, the transition to the triplet state , which assumes an energy level between the first singlet state and the ground state, takes place with a certain probability with less heat emission . This long-lived but reactive state can regenerate chlorophyll in its basic state through further heat emission or phosphorescence or through energy transfer to carotenoids .
Triplet chlorophyll can also transfer its energy to oxygen, which creates reactive singlet oxygen. This highly reactive oxygen species forms peroxides with double bond systems and can thus cause photoinhibition (light inhibition). With medium-term exposure to light, this process causes a long-term effective reduction in CO 2 uptake.
Fluorescence measurements
: Basic fluorescence (minimal fluorescence, constant fluorescence or dark fluorescence). This fluorescence occurs after the weak measuring light is irradiated; it comes from chlorophyll a molecules of the antenna. It is assumed that the excitation energy (excitons) does not reach the reaction centers. Therefore all P 680 reaction centers are “open” (dark adapted), i. H. the associated electron acceptor Q remains oxidized.
: The maximum fluorescence is caused by a short saturation light pulse in addition to the measuring light. All reaction centers P 680 are in a “closed” state, all associated electron acceptors Q are completely oxidized.
and : The variable fluorescence is triggered by switching on the actinic continuous light (Kautsky effect). The variable fluorescence (Fv) is created by actinic light at the reaction centers of photosystem II. It initially increases to peak fluorescence (Fp), the electron acceptor Q is correspondingly partially reduced. When the electron transport starts up on photosystem I and NADP +, the variable fluorescence decreases again. A second, lower maximum occurs when the metabolic cycles that use NADPH start . In the steady state (steady state) of photosynthesis, after about 30 minutes, Fv is constant.
: (Fv) m results from the difference between the maximum fluorescence and the basic fluorescence.
: In the presence of continuous light and ongoing electron transport, saturation pulses produce a reduced fluorescence yield. Since, as a result of saturation pulses, all electron acceptors Q are present in a reduced form, fluorescence quenching occurs here in a non-photochemical way. (Fv) s reaches steady state parallel to Fv.
All fluorescence measurements are given in relative fluorescence units.
Derived fluorescence parameters
Photochemical fluorescence quenching ("photochemical quenching") is a measure of the activity of the electron flow over photosystem II and is calculated as .
Non-photochemical fluorescence quenching ("non-photochemical quenching") is a measure of the reduction in fluorescence that is independent of the electron flow through photosystem II, calculated as .
Analogous to the above equations, two further fluorescence quenching mechanisms were defined:
The coefficient , calculated as , is a measure of the reduction in fluorescence due to mechanisms that prevent some of the reaction centers from being excited by actinic light.
The coefficient is a measure of the reduction in fluorescence caused by actinic light due to electron transport processes, calculated as .
Mechanisms of fluorescence quenching ("quenching")
Processes that reduce fluorescence are known as fluorescence quenching; Under normal environmental conditions, only a few percent of the radiated light energy is emitted as fluorescence. Fluorescence quenching processes reduce the number of excited chlorophyll molecules in the first singlet state and thus also reduce the number of chlorophyll molecules in the triplet state. In principle, this can be achieved in two ways: By reducing the excitation of chlorophyll molecules in the first singlet state or by increasing the dissipation of energy from the first singlet state. All processes that affect light absorption and light conduction (exciton transfer) have an effect on the formation of stimulated chlorophyll in photosystem II. The alignment of the light-absorbing system in the leaf to the light source (Para heliotropism ) influences the quantum utilization of photosynthesis.
In the chloroplasts , a shift of the outer, mobile antenna complex away from photosystem II, out of the grana areas into areas of the stroma where photosystem I is present, can reduce the fluorescence, since photosystem I does not fluoresce. The mobile antenna complex changes its composition. This “photosynthetic control” is triggered by the redox state of the electron transport chain; a similar process, the so-called "spillover" of excitation energy from photosystem II to photosystem I, is not based on the shift of the antenna complex, but on the availability of magnesium in the chloroplast.
The fluorescence can also be reduced by "high-energy-quenching" or "pH-dependent quenching". This non-photochemical quenching of the fluorescence is linked to the inhibition of ATP formation at the ATPase; regulation may take place via the concentrations of ADP , phosphate or reduction equivalents ( thioredoxin ). The rise in the proton gradient across the thylakoid membrane causes feedback, which in the photosystem II and possibly also in the cytochrome b / f complex releases heat.
When the fluorescence decreases, reaction centers arise in photosystem II which, in contrast to functional reaction centers, increasingly convert the energy into heat. A pH-dependent oxidation of chlorophyll has been described as the cause; Furthermore, after an excess of light energy, the three-phase course of the relaxation kinetics of the fluorescence suggests that the pH-dependent xanthophyll cycle contributes to the devaluation of the excess light energy in some plant species.
The conversion of light energy into heat can also be caused by the inhibition of the splitting of water. The exciton transfer and the electron transfer from the water during the excitation or regeneration of P 680 take place at the same speed as the electron transfer to the acceptor Q. When water splitting is inhibited, positively charged P680 accumulates.
Under normal circumstances, photochemistry is a major contributor to the quenching of fluorescence. The energy of the first singlet state is used to transfer electrons to the oxidized acceptor Q (quencher). In addition to the linear electron transport from photosystem II to photosystem I, cyclic electron transport around photosystem II seems to be possible.
See also
literature
- D. Ernst et al. E. Schulze: Chlorophyll determination in the field by fluorometry. in: Archives for Hydrobiology Beih. 16, 1982, pp. 55-61
- B. Georgi, E. Schulze u. D. Ernst: Fluorometric chlorophyll estimation of various algal populations. In: Developments in Hydrobiology 3, 1980, pp. 27-32
- H. Kautsky et al. U. Franck: Chlorophyll fluorescence and carbonic acid assimilation, 9. – 12. Message. In: Biochemische Zeitschrift 315, 1943, pp. 10-232
- G. Krause et al. E. Weis: Chlorophyll fluorescence as a tool in plant physiology II, interpretation of fluorescence signals. In: Photosynthesis Research 5, 1984, pp. 139-157
- H. Lichtenthaler et al .: Application of chlorophyll flurosecence in ecopphysiology. In: Radiation and Environmental Biophysics 25, 1986, pp. 297-308
- G. Papageorgiou: Chlorophyll fluorescence: an intrinsic probe of photosynthesis . In: Bioenergetics of Photosynthesis. Academic Press New York 1975
- H. Schneckenburger u. M. Frenz: Time-resolved fluorescence of conifers exposed to environmental pollutants. In: Radiation and Environmental Biophysics 25, 1986, pp. 289-295
Web links
- Development and testing of a flow sensor for determining the algae class using chlorophyll fluorescence (PDF; 1.1 MB)
Individual evidence
- ^ Albert L. Lehninger: Principles of Biochemistry , de Gruyter, Berlin 1987, p. 714
- ↑ GH Krause and E. Weis, Chlorophyll fluorescence as a tool in plant physiology II. Interpretation of fluorescence signals, Photosynthesis Research 5 (1984) 139-157 doi : 10.1007 / BF00028527
- ↑ C. Buschmann and K. Grumbach, Physiologie der Photosynthese, Springer, Berlin 1985, ISBN 3540151451
- ↑ Norman I. Krinsky, Carotenoid protection against oxidation, Pure & Applied Chemistry 51 (1979) 646-660 doi : 10.1351 / pac197951030649
- ↑ B. Röder: Biological importance of activated oxygen species , Biologische Rundschau 25 (1987) 273-284.
- ^ D. Siefermann-Harms, The light-harvesting and protective functions of carotenoids in Photosynthetic membranes, Physiol. Plantarum 69 (1987) 561-568 doi : 10.1111 / j.1399-3054.1987.tb09240.x
- ^ DJ Kyle and I. Ohad, The mechanism of photoinhibition in higher plants and green algae. In: Photosynthesis III. LA Staehelin and CJ Arntzen, eds., Encyclopedia of plant physiology, new series, vol. 19, Springer, Berlin 1986
- ^ C. Critchley, Photoinhibition, Photosynthetica 22 (1988) 133-134
- ↑ Schreiber U., Schliwa, U. and Bilger W., Continuous recording of photochemical and non-photochemical chlorophyll fluorescence quenching with a new type of modulation fluorimeter, Photosynthesis Research 10 (1986) 51-62 doi : 10.1007 / BF00024185
- ↑ Kautsky H. and Franck, U., Chlorophyll fluorescence and carbon dioxide assimilation, apparatus for measuring rapid luminescence changes of low intensity, Biochemische Zeitschrift, 315 (1943) 139-155
- ↑ Thomas Stuhlfauth, Dieter F. Sältemeyer, Stefanie Weinz, and Heinrich P. Fock, Fluorescence quenching in a water stressed C3 plant, Digitalis lanata, Plant Physiology (1988) 86, 0246-0250 doi : 10.1104 / pp.86.1.246
- ↑ Thomas Stuhlfauth, Ralph Scheuermann, and Heinrich P. Fock, Light energy dissipation under water stress conditions, Plant Physiology (1990) 92, 1053-1061 doi : 10.1104 / pp.92.4.1053
- ^ GH Krause, Photoinhibition of photosynthesis. An evaluation of damaging and protective mechanisms, Physiologia plantarum 74 (1988) 566-574 doi : 10.1111 / j.1399-3054.1988.tb02020.x
- ↑ MM Ludlow and O. Björkman, Paraheliotropic leaf movement in Siratro as a protective mechanism against drought-induced damage to primary photosynthetic reactions: damage by excessive light and heat, Planta 1984 505-518 doi : 10.1007 / BF00407082
- ↑ DC Fork, S. Bose and SK Herbert, Radiationless transitions as a protection mechanism against photoinhibition in higher plants and a red alga, Photosynthesis Research 1986 327-333 doi : 10.1007 / BF00391239
- ↑ TO Glazer and A. Mehlis, Photochemical reaction centers: structure, organization and function, Annual Review of Plant Physiology 38 (1987) 11-45 doi : 10.1146 / annurev.pp.38.060187.000303
- ^ R. Bassi, GM Giacometti and DJ Simpson: Changes in the organization of stroma membranes induced by in vivo state 1 - state 2 transition , Biochimica et Biophysica Acta, 935 (1988) 152-165
- ↑ GH Krause, Photoinhibition of photosynthesis - An evaluation of damaging and protective mechanisms, Physiologica plantarum 74 (1988) 566-574 doi : 10.1111 / j.1399-3054.1988.tb02020.x
- ^ GH Krause, H. Laasch and E. Weis: Regulation of thermal dissipation of absorbed light energy in chloroplasts indicated by energy dependent fluorescence quenching , Plant Physiol. Biochem. 26, 445-452 (1988)
- ^ PQ Quick and JD Mills: Changes in the apparent affinity of CF0 -CF1 for its substrates during photophosphorylation , Biochimica et Biophysica Acta 932 (1988) 232-239
- ↑ KP Hangarter, P. Grandoni and DR Ort: The effects of chloroplast coupling factor reduction on the energetics of activation and on the energetics and efficiency of ATP formation , The Journal of Biological Chemistry 262 (1987) 13513-13519
- ↑ H. Strotmann, S. Kleefeld and D. Lohse: Control of ATP hydrolysis in chloroplasts , FEBS Letters 221 (1987) 265-269
- ^ R. Scheibe, NADP + -malate dehydrogenase in C3 plants: regulation and role of a light-activated enzyme, Physiologica plantarum 71 (1987) 393-400 doi : 10.1111 / j.1399-3054.1987.tb04362.x
- ^ GH Krause, C. Vernotte and JM Briantais: Photochemical quenching of chlorophyll fluorescence in intact chloroplasts and algae - resolution in two components , Biochimica et Biophysica Acta 679 (1982) 116-124
- ↑ U. Schreiber and KG Rienits: ATP-induced photochemical quenching of variable chlorophyll fluorescence , FEBS Letters 211 (1987) 99-104
- ↑ U. Heber, S. Neimanis and KJ Dietz, Fractional control of photosynthesis by the Qb protein, the cytochrome f / b6 complex and other components of the photosynthetic apparatus, Planta 173 (1988) 267-274 doi : 10.1007 / BF00403020
- ↑ E. Weis, JT Ball and J. Berry, Photosynthetic control of electron transport in leaves of Phaseolus vulgaris: Evidence for regulation of photosystem 2 by the proton gradient, in: Progress in photosynthesis research, vol. 2, Dordrecht (1987) 553-556 J. Biggins and M. Nijhoff, editors
- ^ E. Weis and JA Berry: Quantum efficiency of photosystem II in relation to energy-dependent quenching of chlorophyll fluorescence , Biochemica et Biophysica Acta 894 (1987) 198-208
- ↑ DT Sharkey, JA Berry and RF Sage, Regulation of photosynthetic electron transport in Phaseolus vulgaris L. as determined by room-temperature chlorophyll a fluorescence, Planta 176 (1988) 415-424 doi : 10.1007 / BF00395423
- ^ JN O'Sullivan TM Wardley and MJ Dalling: A causal link between galaktolipase and chlorophyll oxidase in wheat leaf chloroplasts , Journal of Plant Physiology 131 (1987) 393-404
- ↑ B. Demmig, K. Winter, A. Krüger and FC Czygan: Photoinhibition and zeaxanthin formation in intact leaves , Plant Physiology 84 (1987) 218-224 doi : 10.1104 / pp.84.2.218
- ^ HY Yamamoto, Biochemistry of the violaxanthin cycle in higher plants, Pure & Applied Chemistry 51 (1987) 639-648 doi : 10.1351 / pac197951030639
- ^ A. Hager, The reversible light-induced conversions of xanthopylls in the chloroplast. In: Pigments in plants, 2nd edition, Fischer, Stuttgart (1980) 57-79, FC Czygan editor
- ↑ E. Pündel and RJ Strasser, Violaxanthin de-epoxidase in etiolated leaves, Photosynthesis Research 15 (1988) 67-73 doi : 10.1007 / BF00054989
- ↑ B. Demmig, K. Winter, A. Krüger and FC Czygan, Zeaxanthin and the heat dissipation of excess light energy in Nerium oleander exposed to a combination of high light and water stress, Plant Physiology 87 (1988) 17-24 doi : 10.1104 / pp.87.1.17
- ↑ Govindjee, WJS Downton, DC Fork and PA Armond: Chlorophyll a fluorescence transient as an indicator of water potential of leaves. Plant Science Letters 20 (1981) 191-194
- ↑ JJ PlijterS. E. Albers, JPF Barends, MJ Vos and HJ van Gorkom: Oxygen release may limit the rate of photosynthetic electron transport , Biochimica et Biophysica Acta 935 (1988) 299-311
- ↑ U. Heber, MR Kirk and NK Boardman: Photoreactions of cytochrome b 559 and cyclic electron flow in photosystem II of intact chloroplasts , Biochimica et Biophysica Acta 546 (1979) 292-306
- ↑ C. Neubauer and U. Schreiber: The polyphasic rise of chlorophyll fluorescence upon onset of strong continuous illumination. I. Saturation characteristics and partial control by photosystem II acceptor side. In: Journal of Nature Research C . 42, 1987, pp. 1246-1254 ( PDF , free full text).
- ↑ SW McCauley, A. Melis, GMS Tang and DI Arnon: Protonophores induce plastochinol oxidation and quench chloroplast fluorescence , Proc. Natl. Acad. Sci. USA 84 (1987) 8424-8428