Marcus theory
The Marcus theory (named after Rudolph Arthur Marcus ) takes the place of the Eyring theory in redox reactions without bond formation or bond breaking . Both theories lead to rate equations of the same exponential form. While the changes in the bond between the reactants during the reaction are decisive in the Eyring theory, the solvent ( outer sphere ) plays the central role in these redox reactions (one-electron exchange reactions) . The Marcus theory shows this and allows the calculation of the Gibbs free enthalpy of activation from the polarization properties of the solvent, the size and the distance of the reactants during electron transfer and the free enthalpy of the redox reaction.
Introduction
Chemical reactions lead to material changes. You can e.g. B. lead to the substitution of a group in the molecule or a ligand in the complex , the cleavage of a part of the molecule or a ligand or to a rearrangement . But it can also only change the charge state of the reactants, and these redox reactions seem to be particularly simple in inorganic chemistry with ions and complexes. In the case of ions of transition metals , such reactions can often be observed in terms of color changes, but organic molecules can also discolor when they accept or release electrons, such as the herbicide paraquat (1,1'-dimethyl-4,4'-bipyridinium), which turns blue when electrons are accepted becomes. This is where the alternative name methylviologen comes from. Marcus developed his theory for a type of redox reaction without structural changes. The original papers should be used for the mathematical reasoning.
In a redox reaction, one partner acts as electron donor D, the other as electron acceptor A. So that the partners can react, they have to diffuse together. They form the so-called precursor complex, usually a kinetic, unstable, solvated collision complex that changes into a successor complex through electron transfer, which in turn diffuses apart. For the one-electron transfer this gives the reaction equation
(D and A can carry charges themselves). Here, k 12 , k 21 and k 30 are diffusion constants , k 23 and k 32 are rate constants of activated partial reactions. The overall reaction can be diffusion-controlled if electron transfer is faster than diffusion. Every shock leads to a reaction. If the reaction is activation-controlled, the association equilibrium is established, with electron transfer being the slower step and separation of the successor complex being the faster step.
Redox reactions usually take place in polar solvents, donor and acceptor centers then have a solvation shell , and precursor and sucessor complexes are also solvated. The inner molecules of the solvation shell, which are very tightly bound, in complexes also the ligands, are called the inner sphere. Redox reactions in which this sphere is involved are called “inner-sphere” reactions. The outer sphere consists of the free solvent molecules. In the case of the “outer sphere” reactions, the inner sphere does not change, no bonds are broken or formed.
RA Marcus has dealt with the nature and size of the free enthalpy of activation in redox reactions, more precisely one- electron transfer reactions of the outer-sphere type, and recognized the central role of the solvent. He initially published two papers. Their results are often referred to as the Marcus theory, although Marcus' work later went far beyond it.
The problem
No bonds are made or broken in outer-sphere redox reactions. There is only one electron transfer (ET). A simple example is the Fe 2+ / Fe 3+ redox reaction. In the self-exchange reaction that takes place in an aqueous solution containing e.g. B. contains both FeSO 4 and Fe 2 (SO 4 ) 3 , takes place in both directions with the same measurable gross velocity, the thermodynamic free standard reaction enthalpy (ΔG 0 ) is zero.
From the temperature dependence of the speed z. B. a reaction such as the S N 2 substitution reaction of saponification of an alkyl halide, an activation energy is usually determined, and this in turn is characterized as the energy of a transition state in the reaction diagram. According to Arrhenius and Eyring, the latter is drawn as an energy diagram with the reaction coordinate as the abscissa, which describes the energetically most favorable path from the starting materials to the products. The points of the reaction coordinates are a sequence of combinations of distances and angles between and in the reactants in the course of the formation and / or the breakage of bonds. The maximum in the energy diagram - the transition state - is characterized by a very specific configuration of the atomic nuclei. In Eyring's theory of the transition state, a very specific change in core coordinates is also responsible for crossing the maximum, which is why the oscillation in this coordinate direction is treated as a translation in Eyring's theory.
This reaction path cannot exist in the outer-sphere redox reactions. Nevertheless, one observes an activation energy. The rate constant for the activation-controlled reaction has the same form as Eyring's equation
In is mainly the transition probability and is the free enthalpy for the formation of the transition state.
The model from Marcus
When electrons are exchanged, the charge distribution changes and this has a major impact on the solvent environment, because the solvent molecules align themselves in the field of charges (this is called orientation polarization ), and the atoms and electrons in the solvent molecules are also slightly shifted (atom or electron polarization ). In redox reactions, this solvent polarization has a decisive influence on the size of the activation energy and thus the reaction rate.
Substitution, elimination and isomerization reactions differ from the outer-sphere redox reactions not only in structural changes, but also in that all individual nuclear and charge shifts ( charge transfer , charge transfer, CT) in the reactants take place in a concerted manner on the most energy-efficient reaction path, the core configurations are therefore always in "equilibrium" relative to one another - the energy maximum therefore only occurs in one dimension of the potential energy surface , in all the others there is still a minimum. An example is the S N 2 substitution of the hydrolysis of an alkyl halide, in which a halide ion is displaced by the rear side attack of the OH - ion and which proceeds via a transition state with a five-bonded carbon atom. The reactant systems are so strongly coupled in the course of the reaction that they represent a uniform structure and ultimately an activated complex. The solvent has an influence that cannot be neglected, but only small in comparison.
In outer-sphere redox reactions, the nuclear shifts in the reactants are very small, whereas the solvent is the determining factor. The coupling between donor and acceptor is weak; both retain their identity throughout the reaction. Therefore the electron as an elementary particle can only “jump” as a whole ( electron transfer ET). The electron jump, if it takes place, is much faster than the solvent molecules can move ( Born-Oppenheimer approximation ). The consequence is: for the electron to jump, the core positions of the two reactants and of all solvent molecules must be the same before and after the fast electron jump ( Franck-Condon principle ); and the energy must not change during electron jump either.
The solvent arrangement depends on the charge conditions. If it were to be the same before and after the electron jump, then any correct arrangement for the self-exchange reaction would be realized, for reasons of symmetry alone, by a solvent configuration that was established when half an elementary charge was transferred. At the same time, the precursor and sucessor complex would have the same energy in this solvent environment. In this solvent arrangement, the conditions for electron jump would be met.
Because the electron, as an elementary particle, cannot be divided, it has to be localized either on the source or target atom or molecule. Thus, in the “transition state”, the solvent configuration (would correspond to the transfer of half a charge) and the charge distribution (charge on one of the partners) are not in “equilibrium”. However, this transition state must be reached before the electron jump, and this can happen through thermal fluctuations in the solvent. The generation of the correct solvent configuration and the electron jump are to a certain extent decoupled and no longer happen synchronously. Actually, they have nothing to do with each other. The energy of the transition state is therefore largely a polarization energy of the solvent.
The Marcus Theory
The macroscopic system: two conductive spheres

Based on these considerations, Rudolph A. Marcus developed a classical theory. Your goal is to calculate the polarization energy of the mentioned non-equilibrium state. From thermodynamics we know that the energy of such a can be calculated if one finds a reversible way to get there. Marcus succeeded in doing this.
Four elements are constitutive for the model on which the theory is based: (1) Marcus initially uses a classic, purely electrostatic model, in which the charge (very many elementary charges) can be transferred between two bodies in any proportions. (2) Marcus separates the fast electron polarization P e of the solvent and the slow atomic and orientation polarization P u on the basis of the time constants with which they are set, which differ by powers of ten. (3) Marcus separates the inner sphere (reactant + solidly solvated solvent shell, for complexes: + ligands) and the outer sphere (free solvent outside). (4) Marcus only calculates the outer-sphere energy of the non-equilibrium polarization of the “transition state” in a solvent, which is usually much larger than the contribution of the inner one because of the far-reaching electrostatic forces (cf. the Debye-Hückel theory of electrochemistry) Sphere. To do this, he chooses a route via two reversible charging or reloading steps.
The tool provides the theory of dielectric polarization in solutions. Marcus solves the problem in general for a charge transfer between 2 bodies of any shape with certain volume and surface charges. In the case of the self-exchange redox reaction, the redox pair (e.g. Fe (H 2 O) 6 3+ / Fe (H 2 O) 6 2+ ) is replaced by two macroscopic, conductive spheres of a certain charge state at a certain distance between them a certain amount of charge is to be reversibly exchanged.
In the first step, the energy W I is calculated for the state in which both balls each carry half of the charge to be exchanged. This state can be achieved through the reversible transfer of half the amount of charge from one sphere to the other by transferring this exchange charge from the donor sphere into the vacuum and from there to the acceptor sphere. The spheres charged in this way generate a certain electric field in the solvent in which the total solvent polarization P e + P u is established. On the other hand, the polarization of the solvent now also generates a field component that affects the charge and polarization.
In the second step, the energy W II of the reversible (back) transfer of half the exchanged charge, again via the vacuum, to the first sphere is determined. However , the atomic and orientation polarization P u is retained , only the electron polarization can set itself in the field of the new charge distribution and the now fixed P u . The system is then in the desired state with an electron polarization that corresponds to the initial state of the redox reaction, and an atomic and orientation polarization that corresponds to the activated complex. The energy W I + W II of this state is thermodynamically a free enthalpy G.
Of course, in this classic model, the charge transfer is not only possible for half the amount of charge, but also for other portions Δe. So you can sense the energy as a function of the distribution of the charge on the two spheres and thus the solvent polarization. In this elegant way, Marcus has combined the coordinates of all solvent molecules into a single polarization coordinate Δp, which is determined by the charge transfer Δe, and thus achieved a simplification of the energy representation in only two dimensions: G = f (Δe). The result for two conductive spheres in a solvent is the Marcus formula
where r 1 and r 2 are the radii of the two spheres, R is the distance between them and ε s and ε op are the static and high frequency (optical) dielectric constants of the solvent. Δe is the amount of charge transferred, the graph G vs. Δe is a parabola (Fig. 1). In the Marcus theory, the energy that corresponds to the transfer of a whole charge (Δe = 1) is called the (outer sphere) reorganization energy λ o , i.e. H. the energy of the two-sphere system, in which the polarization corresponds to that of a whole transferred charge, but the charge distribution corresponds to the state before the transfer. The system is symmetrical with respect to the direction of exchange.
The microscopic system: the redox couple
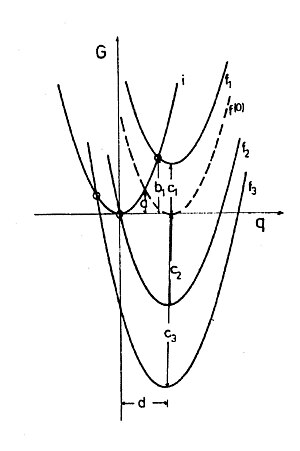
When the classic two-ball system is reduced in size to the point of self-exchange reaction, one arrives at the quantum-determined redox couple in which the charge can no longer be transferred in arbitrary portions, but only as a whole elementary charge. Solvent polarization can still be treated classically, i. E. H. it is not quantized because it is determined jointly by a large number of solvent molecules. Therefore one can calculate the reorganization energy for a hypothetical transfer and retransfer of a partial elementary charge according to the Marcus formula. The reorganization energy is also a parabola for chemical redox systems (Fig. 2.), and it corresponds to free energy or free enthalpy. The energy that the system would have with the hypothetical transfer of half an electron charge (Δe = 0.5) is the activation enthalpy of the self-exchange reaction ΔG (0) ‡ = λ o / 4 (see Fig. 1 and Fig. 2, intersection of Parabolas i and f or f (0)).
Up until now everything was pure physics, now a little chemistry is added. The self-exchange reaction is a specialty among the redox reactions. Most redox reactions take place between different partners, e.g. B.
and thus also show a positive (endergonic) or negative (exergonic) free enthalpy of reaction ΔG 0 .
Since the Marcus calculations refer exclusively to the electrostatic conditions in the solvent (outer sphere), ΔG 0 and λ o are independent of one another and simply additive, i.e. H. the Marcus parabolas of systems with different ΔG 0 are in G vs. Δe diagram just shifted up or down (Fig. 2). A variation of ΔG 0 can be achieved experimentally, for example, by offering different acceptors to a donor.
A simple calculation with the parabolas i (equation y = x 2 ), f (0) (equation y = (x − d) 2 ) and f 1 to f 3 (equation y = (x − d) 2 + c) gives for the free enthalpy of activation
It should perhaps be emphasized again that the point of intersection of the parabolas represents the polarization energy, not the energy of a certain configuration of all nuclei of the reactants, e.g. B. in the mentioned substitution reaction. While the geometry of the transition state in each reactant pair is ideally the same in this case, redox couples with many different polarization environments can meet the energetic condition. For this reason alone, the use of the free activation energy as a thermodynamic quantity is appropriate.
Marcus' formula (2) shows a quadratic relationship between the free enthalpy of reaction and the free enthalpy of activation. It is an experience from the great experimental material of chemistry: reactions usually proceed faster, the more negative ΔG 0 is. In many cases a linear free energy relationship (LFE) is even found. According to the Marcus formula, too, the reaction rates increase when the reactions become more exergonic, but only as long as ΔG 0 is in the positive or moderately negative value range. It is surprising that the free enthalpy of activation for redox reactions in solution according to formula (3) should be greater again if the free enthalpy of reaction is very negative, namely when ΔG 0 is negative and absolutely greater than λ o . This area of the free enthalpy of reaction is called the “Marcus-inverted” area . In Fig. 2 you can see that with a further decrease in ΔG 0 the intersection of the i and f parabolas moves to the top left, which means an increase in the free enthalpy of activation and a decrease in speed. The representation ln k vs. ΔG 0 should therefore be a maximum curve.
The maximum reaction rate is expected at ΔG ‡ = 0. Here also Δe = 0 or q = 0 (Fig. 2). This means that the electron can jump in the equilibrium solvent polarization of the reactants, which is of course much more likely to be realized than a thermally excited one: the reaction is then barrierless. In the inverted area, the polarization corresponds to the difficult-to-think idea of a hypothetical charge distribution on the reactants, in which the donor would have taken up charge and the acceptor would have given up charge . In reality, of course, this does not happen, because it is not a real charge transfer that creates this polarization, but thermal fluctuations in the solvent. The polarization that is necessary for the inverted area can adjust itself with a certain probability due to the thermal fluctuation just as well any other energy of the same kind. In a sense, the electron waits for the correct polarization until it jumps.
The experimental results
Marcus published his theory in 1956. The inverted region was searched for for many years, but the experiments showed only an increase of k up to the diffusion-controlled value for reaction series with decreasing ΔG 0 , i.e. H. to the value at which every collision of the reactants leads to a reaction, and this limit value was also maintained in the region of very strongly negative ΔG 0 values ( Rehm-Weller behavior ). It took about 30 years until the inverted region of Miller, Calcaterra and Closs could be clearly demonstrated, namely in the case of the intra-molecular electron transfer in a molecule in which the donor and acceptor were kept at a fixed distance by a rigid bridge (Fig. 3 ).
Ex post one can assume that with freely diffusing reaction partners the electron jump takes place at the distance R, at which λ o = - ΔG 0 , i.e. ΔG # = 0. Because λ o depends on R, λ o becomes larger with a larger R, the opening of the parabola smaller, and it is formally always possible to narrow the parabolas of Fig. 2 so that the f-parabola passes through the vertex of the i-parabola goes. This means that ΔG ‡ = 0 and the rate constant k assumes a maximum value, that of the diffusion-related limit, for all redox reactions with a very negative reaction enthalpy ΔG 0 . However, there is also the view that a decrease in the speed in the case of very fast electron transfers lies beyond the very negative ΔG 0 values that can be achieved experimentally . The involvement of excited electronic states has also been discussed.
R. A. Marcus and co-workers have refined various aspects of the theory in the years following the theory's initial publication. You have u. a. statistical considerations and quantum effects are taken into account, and they are extended to chemiluminescence systems and electrode reactions. R. A. Marcus received the Nobel Prize in Chemistry in 1992 for his work, and his Nobel Lecture gives a comprehensive overview of his work.
Supporting documents and comments
- ↑ a b c d ELECTRON TRANSFER REACTIONS IN CHEMISTRY: THEORY AND EXPERIMENT. (PDF; 1.0 MB) In: Nobel Foundation. Retrieved April 2, 2007 . or article in "Die Zeit": here
- ^ A b R. A. Marcus: On the Theory of Oxidation-Reduction Reactions Involving Electron Transfer. I. In: The Journal of Chemical Physics . Vol. 24, No. 5, 1956, pp. 966-978, doi : 10.1063 / 1.1742723 .
- ^ A b R. A. Marcus: Electrostatic Free Energy and Other Properties of States Having Nonequilibrium Polarization I. In: The Journal of Chemical Physics. Vol. 24, No. 5, 1956, pp. 979-989, doi : 10.1063 / 1.1742724 .
- ^ Peter W. Atkins : Physical chemistry. 2nd, corrected reprint of the 1st edition. Translated and supplemented by Arno Höpfner. VCH Verlagsgesellschaft, Weinheim et al. 1990, ISBN 3-527-25913-9 , pp. 763-770.
- ^ R. Stephen Berry , Stuart A. Rice , John Ross : Physical Chemistry. Wiley, New York NY 1980, ISBN 0-471-04829-1 , pp. 1147 ff.
- ^ Willard F. Libby : Theory of Electron Exchange Reactions in Aqueous Solution. In: The Journal of Physical Chemistry. Vol. 56, No. 7, 1952, pp. 863-868, doi : 10.1021 / j150499a010 .
- ↑ Marcus places the energy zero point in the vacuum state of the reactants in his calculations. Therefore, many of his equations also contain the solvation energies of the isolated reactants W iso and the electrostatic formation energy of the precursor complex.
- ↑ The quadratic dependence of the outer-sphere reorganization energy is not a consequence of quantized vibrations in the reactants or in the solvent, the theory is purely classical.
- ↑ The reverse reaction can perhaps facilitate understanding: for this, the polarization in the hypothetical retransmission of a whole electron is not sufficient to create a polarization in which the polarization energies of A / D and A - and D + are equal. This situation is only reached with the hypothetical transfer of more than one elementary charge.
- ↑ It is recommended to look at the original work, JACS does not give Wikipedia permission to take over images.
- ↑ Dieter Rehm, Albert Weller : Kinetics and mechanism of electron transfer during fluorescence quenching in acetonitrile. In: Reports of the Bunsen Society for Physical Chemistry . Vol. 73, No. 8/9, 1969, pp. 834-839, doi : 10.1002 / bbpc.19690730818 , have described this behavior by the empirical formula .
- ^ John R. Miller, LT Calcaterra, Gerhard L. Closs: Intramolecular long-distance electron transfer in radical anions. The effects of free energy and solvent on the reaction rates. In: Journal of the American Chemical Society. Vol. 106, No. 10, 1984, pp. 3047-3049, doi : 10.1021 / ja00322a058 .
- ↑ This is supported by the results of studies in which the size of a complex has been systematically changed. (e.g .: Hermann Rau, Rolfdieter Frank, Gerhard Greiner: Rate Dependence of Electron Transfer on Donor-Acceptor Separation and on Free Enthalpy Change. The Ru (bpy) 3 2+ / Viologen 2+ System. In: The Journal of Physical Chemistry, Vol. 90, No. 11, 1986, pp. 2476-2481, doi : 10.1021 / j100402a042 ).
- ^ Paul Siders, RA Marcus: Quantum Effects in Electron-Transfer Reactions. In: Journal of the American Chemical Society. Vol. 103, No. 4, 1981, pp. 741-747, doi : 10.1021 / ja00394a003 ; Paul Siders, RA Marcus: Quantum Effects for Electron Transfer Reactions in the "Inverted Region". In: Journal of the American Chemical Society. Vol. 103, No. 4, 1981, pp. 748-752, doi : 10.1021 / ja00394a004 .
- ^ RA Marcus: On the Theory of Chemiluminescent Electron-Transfer Reactions. In: The Journal of Chemical Physics. Vol. 43, No. 8, 1965, pp. 2654-2657, doi : 10.1063 / 1.1697190 .
- ^ RA Marcus: On the Theory of Electron-Transfer Reactions. VI. Unified Treatment for Homogeneous and Electrode Reactions. In: The Journal of Chemical Physics. Vol. 43, No. 2, 1965, pp. 679-701, doi : 10.1063 / 1.1696792 .