Laser cooling
With laser cooling methods are referred to, with which gas or atomic beams by irradiation with laser light to be cooled. This makes use of the fact that light can transmit an impulse .
The 1997 Nobel Prize in Physics was awarded to Steven Chu , Claude Cohen-Tannoudji and William D. Phillips for the development of laser cooling .
Basic idea
The temperature of a gas is expressed in the (disordered) movement of the atoms, cf. kinetic gas theory : the greater the mean speed of the atoms in a gas, the hotter it is. The speed of the atoms can be reduced by skillfully bombarding them with light quanta ( photons ). If a photon hits an atom, the photon can be absorbed , and a shell electron goes into an excited state . This can “disintegrate” after a while ( spontaneous emission ), a photon is emitted in a random direction (see resonance fluorescence ). Because of the conservation of momentum , the atom receives a small recoil with every absorption and emission .
If you now irradiate atoms with a laser , then each individual atom can scatter a very large number of photons one after the other. The recoil always goes in the same direction during absorption and therefore has a large effect on average over many photon scattering, while the recoil occurring during emission always goes in a different direction and is canceled out over time. By using the Doppler effect , it can be achieved that atoms that are irradiated with laser light from all directions mainly absorb photons from the beam they are moving towards. The resulting force is opposite to the direction of movement of the atoms and slows them down. The mean speed decreases over time, the gas becomes colder.
Doppler cooling
Cooling in the two-state system
A specific transition in the atomic spectrum is used here (often a hyperfine structure transition), which can be viewed as a simple two-state system . By irradiating resonant photons (e.g. laser light) the initial or ground state (see Bra-Ket notation) can be brought into the excited state. After a short time, the excited state will revert to the ground state in two possible ways : on the one hand, stimulated emission is possible, on the other hand, spontaneous emission . In both processes, a photon is re-emitted from the excited atom.
In the case of stimulated emission, there is no net momentum transfer to the atom, since a photon with the same energy is emitted in the direction of the incident photon. However, if the atom spontaneously falls back from the excited state to the ground state , the direction of the re-emission is random. Since the photons had a preferred direction in the radiation, the atoms are slowly accelerated / decelerated in this direction. If you radiate controlled laser light from all spatial directions, you can use it to slow down all atoms - no matter in which direction they are moving. For this purpose, three pairs of two laser beams running in opposite directions are used, which are perpendicular to one another.
Since “hot” atoms move, they are not affected by the frequency of the light in the laboratory system, but by a frequency that has been shifted due to the Doppler effect . You have to emit light that is out of tune with respect to the resonance frequency of the transition in order to stimulate this absorption or reemission cycle. With a certain detuning of the laser light one chooses atoms of a certain speed. This selection is not "sharp" because the atom has an absorption profile in the form of a Lorentz curve and thus a certain line width . If the incident light is redshifted, the process only affects atoms that are moving towards the laser beam.
If, however, two opposing, red-tuned laser beams are used in a spatial dimension , a speed-dependent force results which acts on all atoms and brakes them (see graphic on the right). In order to cool the atoms in question in all three spatial dimensions ( ), six laser beams are used - in opposite pairs. The pairs are perpendicular to each other. This configuration of the laser is as optical molasses (engl. Optical molasses ), referred to because it acts like a viscous liquid and so slows atoms.
The limit of this cooling process is given by the so-called Doppler temperature and is generally in the range of a few 100 μK. The reason for this lower limit are two heating processes that always appear with Doppler cooling. The first is caused by the trembling motion of the atoms due to spontaneous emission. The second is caused by the shot noise of the incident laser light. For rubidium atoms the Doppler temperature is about 140 μK.
This process allows the atoms in question to be cooled, but it cannot hold them in one place. Due to the residual velocity (Doppler limit) they can from the cooling area out diffuse . This is why the method is expanded to include a magneto-optical trap (MOT), in which the atoms are still trapped in a magnetic field and thus remain localized. It can also be used to reach temperatures lower than the Doppler limit.
With the help of the Zeeman effect , the atomic transition in a magnetic field can be detuned so that the Doppler shift is compensated. The so-called Zeeman slower uses this mechanism to slow down a beam of fast atoms.
Real atoms and pumps back
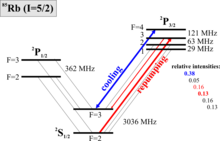
A system of two states is required for cooling, so that a transition is stimulated by excitation with photons , which can only decay into the initial state. In the two-state system this is always given. However, one used in laboratory systems are simply not perfect two-state systems, and so there is always the chance that atoms decay in a state in which they are not resonant to the incident light ( dark state , engl. Dark state ) and so from the Cycle get lost. In the picture on the right you can see the rubidium junction, which is used for cooling because it is in the near infrared (approx. 780 nm) and lasers of this wavelength are readily available. The excited state of the cooling transition (drawn in blue) breaks down once every 1,000 cycles into the lower 2 S 1/2 state F = 2 instead of F = 3 , from which it is brought back with a pump back laser (often casually referred to as a "repumper") must become. The decay does not happen directly because the transition from F = 4 to F = 2 is forbidden ( F = 0, ± 1 , see selection rule ), but the laser also excites due to the very large line width of the atomic transition (approx. 10 MHz) the 2 P 3/2 , F = 3 state, which can then decay into the lower ground state. The pump back laser lifts atoms from the lower ground state to 2 P 3/2 , F = 3 (see drawing), from which they can then return in the intended cycle (decay shown in thin red).
This fact is used to condense the atoms in a so-called dark spot MOT more than would normally be possible.
Subdoppler cooling
There are several ways to cool an atom below the Doppler temperature. In a magneto-optical trap, a moving atom in an optical standing wave is periodically pumped back and forth between different magnetic sublevels, converting kinetic energy into potential energy. This so-called Sisyphus cooling is more effective than Doppler cooling at low speeds and can cool atoms to the limit of their recoil. This limit corresponds to the energy that an atom at rest takes up when it absorbs a photon.
Atoms can be cooled below the recoil limit by ensuring that once resting atoms no longer interact with the laser light. This is z. B. achieved in that the atoms are cooled in a speed-dependent dark state . This method is called VSCPT from the English velocity selective coherent population trapping .
Since the Doppler temperature only depends on the spontaneous scatter rate, i.e. on the lifetime of the excited state, one can use some atoms, e.g. B. with alkaline earth metals , even by a second Doppler cooling step on a very long-lasting intercombination transition to achieve extremely low temperatures.
The method of evaporative cooling is used to achieve even lower temperatures, at which the quantum mechanical degeneration of the atomic gas can be observed .
Sideband cooling
Sideband cooling is a laser cooling method that is related to Doppler cooling, but which is mostly not applied to atomic gases but to individual ions in an ion trap . One exploits the fact that the particles cannot move freely in such a case, but can only occupy discrete vibration states. In this case, the ion can not only absorb light at its resonance frequency, but also at frequencies whose distance from the resonance frequency corresponds precisely to the energy distance between neighboring vibration levels. These are known as the blue and red sidebands . These sidebands roughly correspond to the Doppler shift in the case of laser cooling of free atoms. In the simplest case, an ion is excited on the red sideband, and its vibration excitation is reduced by a quantum . The excited state of the ion then decays spontaneously, with transitions at the resonance frequency or on one of the sidebands being possible. The ion is then back in its ground state, but has (with a high degree of probability) lost vibration energy. Ideally, this cycle repeats itself until the ion reaches the lowest possible vibration state. This can be recognized experimentally from the fact that there is no longer any fluorescence on the red sideband. In practice, optical transitions are generally used in which the excited state has a very long life. Therefore, it is not possible to wait for the excited state to spontaneously decay. One or more pump back lasers are then used, which can bring the ion back to the ground state more quickly via another intermediate state.
References and comments
- ↑ Harold J. Metcalf, Peter van der Straten: Laser Cooling and Trapping , Springer Verlag, 1999, ISBN 0-387-98728-2 , pp. 58-59
- ↑ Of course, the stimulated emission also leads to a trembling movement, but it does not produce any net deceleration because the two emitted photons point in the same direction as the previously absorbed photons. Therefore one can assume that only the spontaneous emission leads to the temperature limit.
literature
- William D. Phillips : Laser cooling and trapping of neutral atoms. In: Reviews of Modern Physics. 70, No. 3, 1998, pp. 721-741, doi : 10.1103 / RevModPhys.70.721 .
- Harold Metcalf , Peter van der Straten: Laser Cooling and Trapping , Springer Verlag, 1999