Updraft power plant

In an updraft power plant (sometimes also called thermal power plant ), air is heated by the sun and rises in a chimney due to natural convection . One or more turbines generate electricity from this air flow .
The principle was described in 1903 by the author Colonel Isidoro Cabanyes in his article in the magazine La energía eléctrica and patented by Bernard Dubos in 1929. In 1931 the author Hanns Günther described a updraft power plant in his book In Hundred Years .
At the end of the 1970s, the idea was taken up by Michael Simon, who designed a pilot system together with the Stuttgart civil engineer Jörg Schlaich , which was then implemented in Manzanares (Central Spain) as a research project of the Federal Ministry for Research and Technology . For several years it showed the technical feasibility in practical operation, but only on a small scale.
function
The sun shines through a large roof made of glass or translucent plastic or foil ( collector ) and heats the ground and the air below. This warm air is forced into a chimney in the middle of the system by the static buoyancy of the warmer air (with a lower density ) . There is an updraft ( thermal ), i.e. H. the lower density of the warm air column in the tower compared to the outside air results in a pressure difference that can be used mechanically. The pressure conversion by the turbine (s) can take place at the bottom in the circumference of the entrance to the chimney tube or (as in the prototype) by a vertical axis turbine at the lower end of the tower tube. The turbines convert the mechanical energy into electrical energy via generators .
The main parameters for the performance of a solar chimney power plant (AWK for short, SCPP for Solar Chimney Power Plant ) are the area of the collector for converting the radiation into heat and the height of the chimney for converting this heat into a pressure difference. The larger the covered area, the more air is heated, and the higher the chimney, the greater this usable pressure difference.
Dependencies
Chimney power plants are dependent on site parameters and local weather conditions. The large area required by solar thermal power stations and the most important of the weather parameters, global radiation, suggest locations in arid zones (e.g. the Sahara) without real estate acquisition costs. The table lists influences from meteorological and geographical variables, also in comparison with other types of regenerative power plants.
variable | Type | impact | Comparison / comment |
---|---|---|---|
Global radiation | meteorology | When the sky is overcast: the diffuse radiation can also be used by updraft power plants. | Concentrator systems (trough, tower and Stirling Dish power plants) can only utilize direct radiation from the sun. |
Outside temperature | meteorology | Low site air temperatures (both on the ground and in higher air layers) increase the tower efficiency. | Improvements in efficiency through low temperatures are also possible with photovoltaic cells (PV). |
Wind speed | meteorology | Negative: lower collector efficiency due to increased convection losses to the environment in strong winds. Positive: Cross winds at the top of the tower can create additional negative pressure and thereby increase performance. Losses predominate, locations with less wind are preferable. | Complementary location selection to wind turbines |
relative humidity | meteorology | The water vapor-air mixture is lighter than dry air. This results in an efficiency improvement when the water vapor content in the tower is greater than outside. In the case of very high input moisture or evaporation in the collector, condensation in the tower is possible. The additional heat of condensation that then arises increases the lift (see thunderclouds) | Targeted condensation to obtain water is conceivable for locations near the sea. |
Precipitation | meteorology | When it starts to rain, the output of updraft power plants first increases, due to the drop in air temperature in the surrounding area, while the working air is further heated from the collector base, similar to the night output. Rainwater that has penetrated the collector base has a performance-reducing effect due to evaporation losses. | In non-concentrating solar systems (AWK, PV), rain has a cleaning effect on the collector surfaces. |
Air pressure | Meteorology / location | High air pressure improves efficiency. | Very high locations (over 1000 m above sea level) are disadvantageous for AWKs. |
Atmospheric counter radiation | Meteorology / location | High IR radiation reduces long-wave emission losses (improves the collector's greenhouse effect). | In all solar power plant types with hot surfaces, the radiation losses follow a T 4 law. Except for AWKs, the meteorological variance of the atmospheric counter-radiation has no noticeable influence on these losses. |
Soil condition | Location | The color of the floor is decisive for the degree of absorption of the radiation, the storage capacity of the floor for night performance is u. a. depends on its density, conductivity and specific heat capacity. Processing of the collector base may be useful. | In the case of other types of solar power plants, no influence of the soil properties is known. |
Tower radius | Plant geometry | The large tower area reduces friction pressure losses in the flow and helps to keep the temperature lift in the collector and thus collector losses low. | |
Tower height | Plant geometry | A greater height of rise improves the tower efficiency and thus also achieves an improvement in the collector efficiency with a larger mass flow and a smaller temperature lift in the collector. | |
Collector radius | Plant geometry | It determines the amount of radiation that can be collected and thus also the electrical output. Enlargement of the collector also requires an increase in the tower, as otherwise the air in the collector will be too slow and too hot and greater losses (convection and IR emission) and thus lower collector efficiency must be expected. |
AWKs are an alternative to wind turbines at level locations with little wind, and at locations with a high degree of coverage they are also a way of using the diffuse radiation components in contrast to the concentrating solar systems. The suitability for solar power generation at locations with the typical combination of "low wind location / location with high degree of coverage" is otherwise only covered by photovoltaics . This has the advantage over AWKs that it can be expanded in modules (starting with small units and a correspondingly low initial capital investment), but until 2007 was at least twice (up to a maximum of 8 times) as expensive as updraft power plants in the 200 MW range.
particularities
AWKs can also generate electrical energy at night as the ground warms up during the day. At night it releases this heat energy again and can continue to heat the air under the collector. Because the ambient air is cooling down at the same time, there is still sufficient lift to operate the power plant. With the appropriate soil condition or special measures to increase the storage capacity of the soil (e.g. black water tank), the daily performance profile can be designed to be even more balanced: The increased intermediate buffering of heat in the ground during times of high noon irradiation lowers the performance maxima when the sun is at its highest, but increases it instead the share of night output when this heat is returned to the working air. The daily course of the supply of electrical power through AWKs is always flatter and wider compared to the daily production curve of other solar power plants, which follow the course of the current solar supply, which therefore generally have a pronounced midday peak and a sharp drop in power towards sunrise and sunset (see diagram "Daily cycle of power and energy from an updraft power plant and a photovoltaic system").
At the test facility in Manzanares, in addition to night performance, another special feature of AWKs could be observed: considerable increases in performance with approaching cold or rain fronts. In addition, the test facility succeeded in causing the air in the tower to rotate like a tornado by generating a pre-swirl in the canopy. A continuation of the vortex beyond the top of the tower to greater heights, as one wants to use in hypothetical air vortex power plants to generate energy, could not be observed.
The method of how solar energy can be made affordable and competitive is based on the same idea with AWKs as with concentrating systems: You replace expensive high-tech components for converting sunlight (e.g. solar cells) with very large, but extremely inexpensive ones Collectors (glass mirrors, channels, greenhouse) and combine these with a centrally placed converter (Stirling or tower receiver, oil-containing absorber pipes, tower). The most striking feature of AWKs compared to other types of solar power plants is the simple relationship between the physical efficiency and the geometric dimensions of the systems over several orders of magnitude.
power
The overall efficiency for the achievable electrical power related to the solar irradiation on the collector is very low with values below 1% - compared to the technical elegance and the high efficiency of photovoltaic systems , the principle of “updraft power plant” seems to be hopelessly subject to. The fact that the solar efficiency does not have to be a measure of the profitability of a power plant is shown by the hydropower plants: They work economically and nobody would think to doubt this because of their low solar efficiency - and yet the sun is the original engine for them too The way to generate electricity, namely via evaporation, cloud formation, winds to transport the water to the reservoir, with a negligibly small degree of efficiency on the primary solar energy required for this.
The construction and operating costs in relation to the yield are obviously essential for profitability. It should be briefly shown that with their rough, rough formulation in connection with the efficiency, an interesting property of AWKs emerges, which distinguishes them from all other solar power plants:
The peak power of a solar thermal power plant can be expressed as the product of the machine, tower and collector efficiency multiplied by the global radiation incident on the entire collector surface :
The tower efficiency is that of a Joule process that describes the isobaric heating in the collector, based on the outside temperature T a , then the adiabatic expansion in the tower with the subsequent release of heat to the atmosphere at temperature level T ah at height h at the top of the tower. For adiabatic stratification of the ambient air is
,
and it results in the conversion ratio of the (mechanical) pressure energy gained to the thermal energy collected in the collector

The conversion efficiency of heat into pressure energy is therefore independent of the temperature lift in the collector. A non- adiabatic stratification of the outside air can be described by an empirical, weather and location-dependent correction factor s, which allows the tower efficiency to vary by 1–3% depending on the weather and time of day. The use of the (absolute) virtual temperature T vakelv takes into account the water vapor content of the ambient air. The same result is obtained if the total available pressure difference is calculated from:
- Pressure difference = height difference × density difference (outside – inside) × acceleration due to gravity,
- with a change of sign, the same formula as for hydropower plants. The multiplication of the extracted pressure portion with the mass throughput gives the output for both types of power plants.
The peak performance is then too
and with the abbreviation
the annual peak output will increase
with c 0 as the location-dependent coefficient, with the values from the table above for the Barstow location in the Mohave Desert in California / USA, which in the selected case includes the midday peak of solar radiation (see table below). The same - namely the formulation of the electrical power by only three factors - also applies to the average power if the corresponding radiation mean value is used instead of the maximum. For the geometry of a system as in the table below, c 0 = 0.0052 results in a power peak of 200 MW. With the same c 0 , but the dimensions of the prototype with tower height 195 m and collector radius = 122 m, the result is a power of 47.3 kW, an excellent correspondence with reality, if one takes into account that the absolute maximum power in Manzanares of 51.7 kW was only achieved once during the project's multi-year duration.
With a simple equation with only three factors one is not able to describe the dynamic behavior of an AWK, e.g. B. to optimize a canopy height profile, to simulate the soil storage behavior or to test variants of the turbine area as a model. For such tasks, detailed calculation models specially developed for AWKs must be used, in which all the weather parameters listed in the table above are included as specifications with a temporal resolution of 10 minutes or less.
variable | meaning | typical value |
---|---|---|
Annual maximum of global radiation, design point for the AWK peak power (depending on location) | 1015 W / m² | |
Virtual outside temperature at a height of 2 m; it is used in meteorology to be able to treat the air-water vapor mixture with the gas equation (and is therefore dependent on the relative humidity, location-dependent) | 295.57 K | |
Specific heat capacity of the air | 1005.0 Ws / kg * K | |
Gravity acceleration | 9.81 m / s 2 | |
Correction factor for the tower efficiency to take into account non-adiabatic stratification of the ambient air around the tower (depending on the location) | 1.017 | |
Tower height | 1000 m | |
Tower radius | 60 m | |
Collector radius | 3500 m | |
Collector area = total area tower area | 38.47 km² | |
Collector efficiency = ratio of the absorbed heat output of the air flow to the global radiation radiated onto the collector surface | 0.252 | |
Tower efficiency = degree of conversion of heat to pressure energy | 0.0336 | |
Machine efficiency (turbine blade losses, gearbox losses, generator losses, etc.) | 0.721 | |
Optimized proportion of the pressure extraction through the jacketed turbine, taking into account the feedback on the collector efficiency. Without this feedback, the power would be maximum at a theoretical value of xt = 2/3. The remaining part of the total pressure difference remains in the flow as a dynamic pressure component (outlet loss) | 0.84 | |
Total solar efficiency of the system | 0.005 | |
Abbreviated summary of location values and efficiency levels | 0.0052 1 / m | |
Total construction costs for the tower divided by the tower surface = area-specific tower costs to optimize the geometry within defined limits | 498.7 € / m² | |
Total construction costs for the collector, divided by the collector surface = area-specific collector costs to optimize canopy heights and canopy radius within defined limits | 8.29 € / m² | |
Specific costs for turbine (s), gearboxes, generators, etc., to be applied as proportional to the maximum output | 0.73 € / W peak | |
specific engineering costs of a 200 MW peak system | 0.24 € / W peak | |
specific total investment costs, based on the installed peak power | 3.4 € / W peak |
economics
The example of a 200 MW system shown above has an overall solar efficiency of only 0.5%. If you take a closer look at the efficiency chain, it quickly becomes clear that only one of them can be improved to a greater extent: the collector efficiency - in the example it is only 25.2%, which roughly corresponds to the average achieved in the pilot system. The maximum technically feasible degree of efficiency is around 80% for flat, upward-facing collectors - as is the case with common (water) flat-plate collectors - which can be achieved if the thermal surface losses are minimized through good insulation while keeping the temperatures low (e.g. by means of a small temperature lift of the working air and vacuum insulation of the cover). The total losses would then be reduced to the optical reflection losses.
At the current level of development, however, such collectors would be too expensive, and efficiency improvements would reveal the economic advantage of AWKs - this stands or falls with the low collector - in relation to the remaining costs. A factor 3 in the technical development potential for collector efficiency improvements, combined with corresponding increases in yield and / or area savings, certainly offers sufficient incentives for further research and development work.
On the other hand, it should also be ensured that the efficiency of large systems is also maintained in this order of magnitude and does not decrease below 25%. This can occur e.g. For example, if the towers are too slim: With the same tower height and the same collector area, the same output with a smaller tower radius would only be achieved through a larger temperature lift due to the lower mass throughput - but this causes higher losses, so the slimmer tower always has the lower output. Another critical influencing factor is the height profile of the greenhouse towards the center: a canopy that is too low leads to friction pressure losses at high air speeds. In each size level of AWKs, certain similarities in the geometrical dimensions, material properties and construction methods must be observed, which justify a cost approach of both collector and tower costs as proportional to the respective surface (p. 285 for systems in the 100 MW range) . To determine the total costs per installed “peak” watt (W peak ), you can proceed as follows.
The total construction costs are made up of the machine costs (turbines, gears and generators), the tower costs and the collector costs plus an engineering component. If this is divided by the electrical peak power according to Eq. (6), the peculiarity of AWKs can be seen very clearly from this simple connection, namely the fundamental dependence of the specific investment costs on the geometry of the systems. This connection should be briefly derived.
With the proportionality of the costs to the respective surfaces, the following applies:
and
The machine costs are set in proportion to the installed electrical power (also the engineering costs), whereby the annual maximum output must be covered:
or by inserting values from the table:
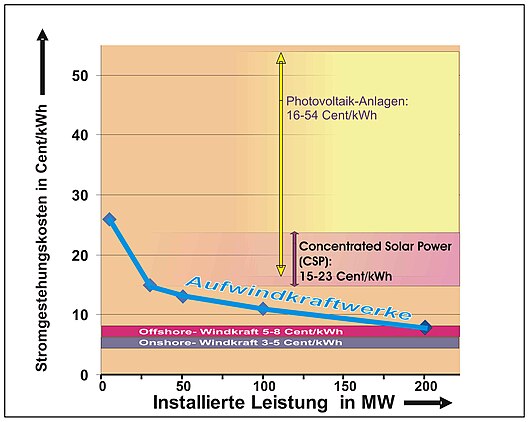
The summands of the specific investments show the following behavior with increasing tower height and increasing collector radius - and therefore increasing output:
- the specific machine and engineering costs remain constant (and at a low level compared to the other two components).
- the specific tower costs in the second term are independent of the tower height and inversely proportional to the collector area, i.e. H. they become smaller with increasing output and are less important compared to the collector costs.
- the specific collector costs in the third summand also fall in principle with increasing system dimensions: they are inversely proportional to the tower height.
This behavior of AWKs is not to be confused with a cheaper component through series effects or through large-scale industrial production: this latter effect occurs in general with all types of power plants and can be with AWKs - e.g. B. when building several systems at nearby locations - additional costs. This dependency on costs, performance and geometry is also valid regardless of the fact that the specific investment costs of AWKs are not a good measure for comparing solar power plants with one another because of the flattened daily profile of performance.
With the location values from the table, these formulas give the annual peak power of 200 MW and the specific investment costs of 3.27 EUR / Wpeak or 3270 EUR / kWpeak. The average capital costs per kW of installed capacity in solar power plants amount to 4000 EUR / kWpeak, with AWKs not being taken into account. According to this comparison, AWKs of this size are cheaper to build than all other solar power plants, and this is all the more true as the peak output in the denominator of specific costs at the other power plants has a considerably higher ratio to the average daily or annual output than with AWKs. Therefore, especially with AWKs, the electricity production costs are to be used to assess the profitability instead of the capital costs. Here the authors of the research report in 1985 named a value around (converted) 13 cents / kWh. The value given by the planners of the current projects (see below) in 2007 is 8 cents / kWh for a 200 MW system (p. 71). For comparison: The electricity generation costs of concentrating systems (trough power plants, tower power plants and Stirling Dish systems) are between 15 cents / kWh and 23 cents / kWh depending on the places where there is higher and lower solar radiation, those of photovoltaic systems at 16–54 cents / kWh called.
It is common practice that when considering the costs of research and development and the first-time custom-made project components (at AWK Manzanares: turbine, gearbox and two generators, tower made of trapezoidal sheet metal with supporting framework, canopy with 4 different test covers) the Far exceed the costs of series production and always make the projection into the “promised” profitable future seem somewhat questionable. This is not the case with the updraft power plant: if the peak output of 51.7 kW achieved is included in the above formula, with specific investment costs of 67,307 EUR / Wpeak, a construction cost of 3.2 million EUR is obtained - i.e. considerably less than the pure construction costs of the prototype, which are quoted at 3.7 million DM for the tower and canopy. This is due to the fact that the specific costs of a concrete tower, as planned for large-scale systems, cannot be compared with those of a trapezoidal sheet tower as in the pilot system.
Neither the performance nor the specific investment costs can be clearly defined by equations (6) and (10a), even if one disregards the weather and location uncertainties. This is due to the fact that a low, easily achievable fixed collector efficiency was assumed. The reality is that structural variants, but also changes in the system geometry alone, can affect the collector efficiency in the order of ± 30%. The actual engineering task, as almost always, is to find the most economical compromise between "structurally feasible" and "physically sensible". In particular, increased friction losses in the canopy and tower at updraft speeds of 15 m / s and higher have to be simulated in detailed flow models.
So updraft power plants seem to be a promising alternative for our solar power supply - since, according to the above considerations, they function optimally in areas with little wind but sunny areas, they are a sensible addition to wind power plants there. Because of the simple principle, there are hardly any technical uncertainties; they are of the order of magnitude of the weather uncertainty. The prototype in Manzanares was already certified by the technical advisors of the Research Ministry from the KFA Jülich as demonstration character, which made further costly research projects unnecessary.
The fact that no further AWK has been completed since the Spanish pilot project, although there was and is talk of a start of construction again and again, reveals the weakness of the principle: the minimum size of the plants in the 100 MW range, which is necessary for economic viability, combined with the corresponding high cost of capital. The step from a 50 kW system directly to a 200 MW power plant, without any further intermediate steps, seems risky to some investors, the existing large systems such as Andasol with 150 MW after completion can look forward to a long development period with a strong increase in know-how look back and could be expanded step by step - and that is not possible with AWKs.
Land use and environmental impact
For efficient operation, updraft power plants must have a corresponding size both in the collector radius and in the chimney height. This results in a large amount of land being used. The area under the roof could in principle be available for other uses, e.g. B. as a greenhouse in the classic sense for plants that need heat. Agricultural tests on the prototype, however, resulted in rapid withering of the test plants; with appropriate irrigation, this resulted in a loss of performance due to evaporation or a reduction in the transparency of the roof due to condensation.
The fear that a large number of AWKs could have consequences for the climate speaks against the fact that the natural thermals that are used here are an integral part of all weather events and all climate processes and at the same location to the same extent, only with a time lag, even without Collector and chimney occurs. The strong dependency on the local weather as well as the geological characteristics of the location speak more for a strong environmental impact on the system and its performance than for a reaction in the other direction. This includes in particular the influence of - depending on the location - possible sandstorms. The decrease in transparency due to dusting has proven to be uncritical in the pilot plant, but a closed deposit of sand would have to be removed actively or even automatically. How this could be done and what effects such measures have on construction and maintenance costs have not yet been examined in detail. Likewise, the possible causes and effects of the frequent occurrence of tinged winds above the collector roof, as observed on the prototype, have not yet been investigated.
Manzanares pilot project
Coordinates: 39 ° 2 ′ 34 " N , 3 ° 15 ′ 12" W
Half a century after Hanns Günther's future design, Jörg Schlaich from Stuttgart developed his updraft power plant and built a first test facility with a peak output of 50 kW on behalf of the German Federal Ministry of Research in Manzanares (Central Spain). The test facility in Manzanares had a collector radius of 122 m and a chimney height of 194.6 m. This gave it an output of 50 kW .
The work began in the spring of 1981 under the direction of the engineers from Schlaich + Partner, five fitters and ten Spanish unskilled workers from the Munich company Maurer Söhne , who were experienced in building thin-walled chimneys . A ring was installed on eight inclined pipes at a height of 10 m, and another ring on the floor, which could be pulled up to the support ring via hydraulics . A pipe with a diameter of 10 m was assembled piece by piece from 1.2 mm thick, trapezoidal sheets on the lifting ring on the floor. The first eight meter high section of the chimney was now lifted up to the support ring on the lifting ring, then the next eight meter high section was put together. The 250-ton chimney was erected in this “eight-meter cycle”, with outer rings every four meters reinforcing the tube. On some of the reinforcements, 4 cm thick bracings with permissible load-bearing forces of 50 t each attack, which stabilize the tower in a star shape in three directions. For reasons of cost, massive DYWIDAG rods were used instead of wire ropes , as are known from bridge reinforcement. They were anchored in concrete foundations and provided in the lower “1/10 point” with vertical bracing to dampen vibrations (photo: silhouette with foundation and bracing in front of the night sky).
In the summer of 1981, the assembly of the foils began, which were stretched two meters above the ground on supporting frames with fields of 4 m × 6 m and 6 m × 6 m, in the middle of the fields there is an approx with a drainage hole for the rainwater (photo: still intact fields in the foreground). Half of the system consists of 0.1 mm thick, particularly strong polyester films with UV protection from Kalle / Hoechst . The other half consists of 0.1 mm thick Tedlar foil based on fluorine with a lower mechanical strength. After the initial storm damage to the foils (photo), stable yet inexpensive glass greenhouse covers were also successfully tested. In late summer, the company Balcke-Dürr / Ratingen installed the turbine and machine system. The wind turbine consists of four glass fiber reinforced plastic blades, which are connected to a generator to generate electricity via a 1:10 gearbox. As soon as the wind speed reaches 4 m / s, the wind turbine starts up; with mains operation the speed is kept constant at 150 revolutions per minute. At lunchtime, the speed increases to over 20 m / s, but is slowed down to 12 m / s by the turbine. After sunset, the floor under the foil roof has stored so much heat that, in the best case scenario, operation can continue all night.
The plant was commissioned on June 7, 1982. From 1983 to 1986, a large number of experiments and optimizations were carried out on the plant. The system was designed for a trial period of three years and should then be removed without a trace.
From the middle of 1986 to the beginning of 1989 the system ran almost without any problems for 32 months. The power plant supplied electricity in 8611 operating hours (approx. 8.9 hours per day). The availability during this time was over 95 percent. In 1987, 44.19 MWh of electricity were generated. This also allowed the theoretical calculations, which were based on a yield of 44.35 MWh, to be fully confirmed.
The technical feasibility of such a system on a small scale, which was often questioned until then, could thus be demonstrated. In 1989 the plant fell victim to a storm lasting several days.
Follow-up projects
On December 10, 2010 , the 200 kW Wuhai updraft power plant went into operation in Jinshawan, Inner Mongolia , People's Republic of China at 39 ° 46'2 "N 106 ° 49'48" E. With the last expansion stage, a total output of 27.5 MW should be achieved in 2013. The project was supported by the Ministry of Research and Technology of Inner Mongolia and developed by the University of Research and Technology of Inner Mongolia and the Technical University of Madrid. The construction cost of $ 208 million will be borne by a local company. According to Rudolf Bergermann von Schlaich, Bergermann und Partner, however, the system shows considerable design flaws. The tower is not high enough and the collector area is too small. The collector surface consists of metal-framed panes of glass, many of which have cracked or splintered due to heat stress.
The first planned commercial power plant, the Buronga updraft power plant, was planned by EnviroMission Limited in Australia , near Mildura, from 2005 onwards. The chimney should be 1000 m high, have a diameter of 130 m and be surrounded by a 38 km² collector (7 km diameter). The maximum output was planned to be 200 MW. The project was supposed to start in 2005, but the operator was unable to secure the necessary financing for the construction until 2007. This made the realization of the project unlikely.
In 2007 the physicist Wolf-Walter Stinnes announced that he and his company Greentower near Arandis in Namibia wanted to build a plant with almost 38 km² of greenhouse area (7 km in diameter) and a tower over 1,500 m high. With 32 turbines and a nominal output of 400 MW , the entire electricity demand of the country (excluding large industrial customers) should be covered. The Ruhr University Bochum was also involved in the development. Information about a realization has not become known.
See also
- Air purification tower Xian , a system based on this principle for air pollution control
- Fall wind power plant, a theoretical type of power plant to use an artificially generated fall wind
literature
- J. Schlaich, R. Bergermann, K. Friedrich, W. Haaf, H. Lautenschlager: Planning and construction of a demonstration system for an atmospheric thermal updraft power plant ready for construction. Application-oriented design of larger units and extended measuring program BMFT-FB-T 86-208. Stuttgart 1986.
- Jörg Schlaich: The updraft power plant. Deutsche Verlags-Anstalt, Stuttgart 1994, ISBN 3-421-03074-X .
- Schlaich, Bergermann, Schiel, Weinrebe: updraft power plants for solar power generation. Affordable - inexhaustible - global. CD-ROM with booklet. Bauwerk Verlag, Berlin 2004, ISBN 3-934369-51-0 .
- Jörg Schlaich, Gerhard Weinrebe: Electricity from hot air: the updraft power plant. In: Physics in Our Time. 36 (5) 2005, ISSN 0031-9252 , pp. 212-218.
- Marco Aurélio dos Santos Bernardes: Technical, economic and ecological analysis of updraft power plants. Research report, Institute for Energy Economics and Rational Energy Use (IER), University of Stuttgart, Stuttgart 2004. (online)
Web links
- Storm in the tower. In: Image of Science
- www.enviromission.com.au - plans to build several updraft power plants in Australia
- Achmed AW Khammas: Book of Synergy
- 2nd International Conference on Solar Chimney Power Technology (English)
Individual evidence
- ↑ updraft power plant. ( Memento of March 4, 2016 in the Internet Archive ) Presentation by Farrenkopf, Heinrich, Kuhn, Stenglein on November 10, 2009 Augsburg University of Applied Sciences.
- ^ A b c d e J. Schlaich, R. Bergermann, K. Friedrich, W. Haaf, H. Lautenschlager: Planning and construction of a demonstration system for an atmospheric thermal updraft power plant ready for construction. Application-oriented design of larger units and extended measuring program BMFT-FB-T 86-208. Stuttgart 1986.
- ↑ a b c d e f g SBP table: updraft power plants dimensions and costs using the example of a sunny emerging country ( memento of the original from April 29, 2014 in the Internet Archive ) Info: The archive link was automatically inserted and not yet checked. Please check the original and archive link according to the instructions and then remove this notice.
- ^ A b c I. Werenfels, K. Westphal: Solar power from North Africa framework conditions and perspectives. SWP Study Foundation Science and Politics. German Institute for International Politics and Security, Berlin 2010, ISSN 1611-6372 , p. 26.
- ↑ Introduction to the meteorology physics of the atmosphere. Volume 1, (= BI university paperbacks, volume 276). BI-Wissenschaftsverlag, Mannheim 1973, ISBN 3-411-00276-X , p. 30 ff.
- ↑ Greenpeace International / SOLAR PCAES / ESTELA, Concentrating Solar Power 2008, p. 67.
- ↑ Wolfgang Knapp: Upwind with the sun. In: Image of Science. Issue May 1982, Volume 19, pp. 48–56.
- ↑ energize: Towers of power - the solar updraft tower ( Memento from April 12, 2014 in the Internet Archive ), September 2008
- ↑ Jörg Schlaich: The updraft power plant. Deutsche Verlags-Anstalt, Stuttgart 1994, ISBN 3-421-03074-X .
- ^ Official government website of China - China's first solar chimney plant starts operating in desert. accessed on March 23, 2014.
- ^ The updraft power plant Schlaich Bergermann & Partner: The updraft power plant, accessed on November 27, 2015.
- ^ National Geographic-Solar Chimneys Can Convert Hot Air to Energy, But Is Funding a Mirage? accessed on May 2, 2014.
- ↑ EnviroMission Limited - website called, May 19 2012th
- ↑ Photo series - The tallest buildings in the world , on: sueddeutsche.de , March 28, 2007, accessed May 19, 2012.
- ↑ updraft power plants - technically simple and environmentally friendly. on: Abendblatt.de May 22, 2007, accessed May 19, 2012.
- ↑ Slim giants. In: ruby. 2008, pp. 54–56, accessed May 19, 2012. (PDF)