Smooth musculature
Smooth muscle is one of three types of muscles in humans and animals. It occurs in the walls of all hollow organs except the heart, which can "contract", which means that they can reduce their clear width ( lumen ). These are, for example, the blood vessels, the organs of the digestive tract and the respiratory tract. In addition, smooth muscle occurs in various other places such as the skin.
The name of the smooth muscles refers to their microscopic structure: while the skeletal muscles and the heart muscles show visible transverse stripes under the microscope , the smooth muscles do not. In contrast to the skeletal musculature, it cannot be contracted arbitrarily either, but has other control mechanisms , which range from muscle-specific ( myogenic ) mechanisms to control by the vegetative nervous system or enteric nervous system ( neurogenic control ) and to control by hormones , neurotransmitters and other messenger substances . Apart from these similarities, the smooth muscles form a very heterogeneous (different) group that can be divided into further subgroups. For example, one can distinguish the muscles of the individual organ systems from one another (e.g. smooth muscles of the blood vessels, the respiratory tract, etc.), or differentiate phasic smooth muscles (relaxed at rest or rhythmically contracted) from tonic ones (constantly contracted).
embryology
Like skeletal muscle cells, smooth muscle cells are of mesodermal origin except in the iris of the eye .
construction
Construction at the cellular level
Smooth muscle consists of smooth muscle cells that have a spindle-shaped appearance, about two to ten micrometers wide and about 20 (in blood vessels) to 800 µm (in the uterus of a pregnant woman) are long. The elongated cell nucleus is located in the middle, at its ends are most of the cell organelles such as mitochondria , ribosomes or the rough endoplasmic reticulum .
If the smooth muscle cells appear in large numbers, they form dense layers, strands or grid-like systems, depending on the organ. Each cell is surrounded by a membrane, the basement membrane . The cells are connected to one another via protein systems outside the cells ( extracellular matrix ). This connection between the cells ensures that the entire muscle group can exert a force. Some smooth muscle cells also have tiny tendons that they attach to the connective tissue .
In the electron microscope image, the longitudinally oriented, parallel filaments inside the cell, compression zones inside the cell and on the cell membrane and pits ( caveolae ) on the cell membrane are noticeable. In some muscle cells a system of smooth tubes is also found near these caveolae; it is believed that both may be involved in the muscle cell contraction mechanism.
Structure at the molecular level

On the right side you can see how the actin filaments (red) between the myosin heads (green) move towards one another and thus shorten the muscle.
Under the light microscope, the cytoplasm (inside of the cell) appears homogeneous in contrast to the striated muscles, but there are a large number of structures in smooth muscle cells. Two different systems in combination are responsible for the contraction of a smooth muscle cell: the cytoskeletal system and the myofilaments.
Cytoskeleton
The cytoskeleton of a cell is a framework of proteins inside the cell that supports it, gives it its shape and performs other tasks. The intermediate filaments desmin and, in the case of vascular muscle cells, vimentin and non-muscular actin are necessary for the contraction of a smooth muscle cell . These pass through the muscle cell and range from compression zones inside the cell, the dense bodies , to compression zones on the inside of the cell membrane, the attachment plaques , or dense plaques . These compression zones contain α-actinin and plectin and are comparable to the Z-disks in striated muscles.
Myofilaments
The myofilaments form the actual mechanism for contraction ( contractile apparatus ) and, in the case of smooth muscles, are composed of smooth muscle α-actin and isoforms of myosin . They are likely arranged in inclined paths through the cell. The actin filaments are anchored in the dense bodies and dense plaques mentioned . The myosin filaments are in contact with several actin filaments and can bind to these actin filaments with their head region . The special arrangement of the actin and myosin filaments to each other enables the smooth muscle cells to shorten to a third of their original length. In striated muscle cells, the actin and myosin filaments have a regular arrangement, so that typical striations appear in the microscopic image.
The actin filaments of the smooth muscle cells also have contact with integrins in the area of the dense plaques , which span the cell membrane and are connected to proteins of the extracellular matrix on the outside of the cell. This ensures the contact between the muscle cells.
contraction
The contraction mechanism of the smooth muscles essentially corresponds to the muscle contraction of the striated muscles and basically works as follows: The head domain of the myosin, the myosin head, is already bound to an actin filament at rest. The angle between the head domain (heavy chain) and the light chain is 90 °. In addition, the myosin head has bound ATP . If the muscle is now contracted, there is a strong increase in the Ca 2+ concentration within the cell (from about 10 −7 to 10 −6 to 10 −5 mmol / l). This Ca 2+ now binds to the protein calmodulin , which in turn activates the enzyme myosin light chain kinase ( MLKK ). MLKK phosphorylates the myosin light chain, i. H. Phosphate is added to the molecular structure of the myosin. This gives the myosin ATPase activity and consequently cleaves the ATP bound to the head domain. This step provides the necessary energy. The cleavage products, namely ADP and phosphate, leave the myosin head and this folds over, the angle between the heavy chain and the light chain is now only 45 °. In doing so, the myosin head “shifts” the actin filament a little: a movement is created. This movement is called a force stroke. The individual actin filaments were pushed towards one another, so that the length of the muscle was shortened overall, i.e. it is contracted.
In the next step, it is now necessary to break the bond between the myosin head and the actin filament. To do this, a molecule of ATP is bound again, the bond is released, an angle of 90 ° between the heavy and light chain is set again and the mechanism can start all over again. So it is a cycle, the cross-bridge cycle. This cycle allows the power stroke to be repeated over and over again so that the muscle can shorten to the maximum. In the case of smooth muscle cells, this maximum is approximately one third of the original length, which is significantly longer than in the case of skeletal muscle. However, the contraction is also much slower.
The increase in Ca 2+ contraction can be caused by three different mechanisms:
- Electromechanical coupling:
- Electromechanical coupling describes the process by which action potentials lead to muscle contraction. Action potentials represent ion currents which are characterized by the redistribution of certain ions on the cell membrane and which move along the cell membrane. The voltage changes between the space outside and inside the cell. These action potentials can be caused by pacemaker cells or by nerve sites. In any case, such a voltage change leads to voltage-dependent Ca 2+ channels opening and calcium flowing into the cell from outside, which leads to the contraction described above. Such voltage changes can also come about through a long-lasting change in voltage (depolarization) due to a change in the conductivity for potassium ions. One speaks here of the metabolic contraction .
- Pharmaco-mechanical coupling:
- In the case of pharmacomechanical coupling, hormones, neurotransmitters or pharmaceuticals lead to an increase in the Ca 2+ concentration and thus to a contraction. Hormones and neurotransmitters such as noradrenaline can bind to receptors on the cell surface and increase the activity of the enzyme phospholipase C inside the cell. Phospholipase C forms inositol triphosphate (IP 3 ), which in turn binds to the IP 3 receptor, which is located on the sarcoplasmic reticulum (a cell organelle). This leads to the release of calcium from the sarcoplasmic reticulum into the interior of the cell and thus to contraction.
- In addition, the pharmacomechanical coupling can directly change the activity of the MLKK enzyme (Ca 2+ sensitization). For example, the contraction of the blood vessels is regulated by activators of the Rho kinase of the parasympathetic nervous system. An increase in the activity of myosin light chain phosphatase (MLKP), on the other hand, is called Ca 2+ desensitization, since MLKP has the opposite effect to MLKK, namely dephosphorylating myosin and thus preventing the cross-bridge cycle.
- mechanical coupling: stretch-dependent calcium channels can open directly when stretched and trigger an influx of calcium and thus contraction. This is called the Bayliss effect .
Contraction forms
Structural differences and the resulting functional differences make it possible to subdivide smooth muscle tissue into single-unit and multi-unit types. Mixed forms are v. a. frequent in the vascular muscles .
Single-unit type
If several muscle cells are connected to one another by certain proteins, one speaks of muscle tissue of the single-unit type. The proteins form a kind of channel between the cells, the gap junctions ( nexus ), so that ions and messenger substances such as second messengers can quickly get from one cell to another. This leads to an electrical coupling between the cells: if the ion concentration in a cell changes (e.g. within the framework of an action potential), this change is transmitted directly to the neighboring cell and this is also excited. Therefore, the muscle cells of the single-unit type contract almost synchronously.
The muscle cells in such a cell cluster are stimulated by spontaneously active pacemaker cells ( myogenic tone ) and occur in hollow organs such as the uterus or in small blood vessels. However, the frequency of such connections also varies within the organ and changes in the uterus during pregnancy. The activity of the pacemaker cells can be regulated by vegetative nerve cells or hormones.
Multi-unit type

Contractions dependent on the neighboring cells do not take place or only to a very limited extent in the multi-unit type. Instead, each muscle cell is innervated by nerve fibers of the vegetative nervous system, or also regulated by hormones. For this purpose, the nerve cells in the immediate vicinity emit transmitters from varicosities (thickenings) . One speaks therefore of neurogenic tone . Since the distance between the varicosities and the smooth muscle cells is much larger (approx. 50 to 100 nm) than the distance in "real" synapses, one also speaks of the "synapses à distance".
Relaxation
For relaxation ( relaxation ) occurs by a drop in Ca 2+ -mirror caused by the lack of nerve impulses and other exciting events. Calcium is transported by Na + / Ca 2+ antiporters and Ca 2+ - ATPases ( SERCA ) from the interior of the cell back to the extracellular space or the sarcoplasmic reticulum. The Ca 2+ -calmodulin complex dissociates (breaks down) and MLKP dephosphorylates the light chains of the myosin molecule. As a result, the ATPase activity of the myosin heads succumbs and the cross-bridging cycle can no longer proceed.
In addition, the following mechanism for active relaxation is known: The activation of the endothelial nitric oxide synthase releases the messenger substance nitric oxide (NO), a gasotransmitter, in the corresponding vascular section. Nitric oxide (NO), which is constitutively formed by the vascular endothelium, can diffuse into the neighboring smooth muscle cells and activate the soluble guanylate cyclase there . The consecutive increase in the cGMP level leads to an activation of protein kinase G , which activates myosin light chain phosphatase (MLKP) by phosphorylation and thus leads to the relaxation of the smooth muscle cells. The stimulation of the β-adrenoreceptors, for example by adrenaline, leads to an activation of the MLKP and thus to a relaxation of the muscle cell via the increase in the cAMP level and the activation of protein kinase A. (see regulation).
regulation
There are several mechanisms for regulating smooth muscle tone, two of which are shown below:
- Myogenic regulation describes the exciting influence of the pacemaker cells: smooth muscle cells or specialized fibroblast-like cells generate slow depolarization waves that can lead to action potentials. This process can be promoted by stretching via stretch-dependent Ca 2 channels. This type of regulation is found in the single-unit type.
-
Neurogenic regulation requires that almost every muscle cell is innervated . Here regulation takes place via nerve impulses from the autonomic nervous system, which is made up of the sympathetic and parasympathetic nervous systems. In the digestive tract, the enteric nervous system is also involved:
- Sympathetic: The nerve cell bodies of the sympathetic are located in the spinal cord and pull to the paravertebral ganglia of the border cord or to the prevertebral ganglia, where they connect to the second neuron relatively far from the organ via cholinergic synapses. The second neuron ultimately innervates, among other things, the smooth muscles via adrenergic synapses. The effect depends on the receptor: the α1 receptor mediates a contraction of the muscles (e.g. in blood vessels, the iris, the sphincters in the digestive tract and the urinary bladder), while the β2 receptor has exactly the opposite effect: it is activated , the bronchi, blood vessels in skeletal muscles and the heart expand. It should be noted that the effect on the β2 receptor is only caused to a small extent by nerve fibers, because the corresponding muscles are only very inadequately innervated. The stimulation by catecholamines, especially adrenaline and noradrenaline from the bloodstream, is of much greater physiological relevance. All of these effects of the sympathetic nervous system on the organism generally benefit the preparation of the body for greater physical exertion ("fight or flight").
- Parasympathetic: Instead of an organ remote interconnection of pre- to postganglionäres Neuron as in the sympathetic nervous system is with the parasympathetic a relatively organ close interconnection before: The preganglionic fibers from the spinal cord or the vagus nerve pull the Cilarganglion , otic ganglion , submandibular ganglion and pterygopalatine ganglion or ganglia that are in the immediate vicinity of or even in the organ itself. The postganglionic fibers then reach the smooth muscle cells via muscarinic, cholinergic receptors. The M1, M3 and M5 receptors apply to the smooth muscles and cause the bronchial muscles and other muscles to contract. In the vessels, in turn, the effect is explained through the endothelium: Acetylcholine is supposed to bind to M3 receptors on endothelial cells, which in turn release muscle-relaxing substances to the smooth muscle cells.
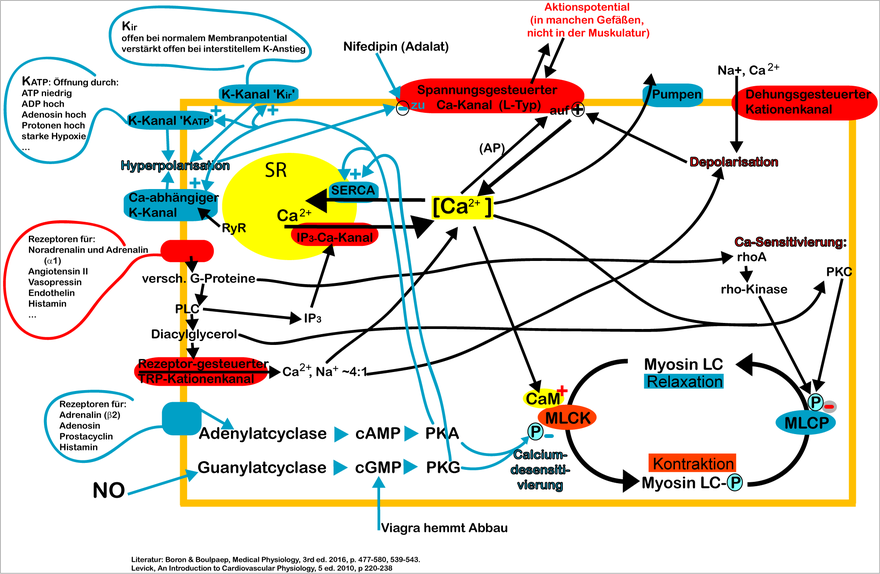
Myosin LC: Myosin light chain. MLCK: myosin light chain kinase. MLCP: myosin light chain phosphatase. CaM: calmodulin. ℗: phosphorylation. PLC: phospholipase C. PKA, PKC, PKG: protein kinase A, C or G. NO: nitric oxide. SR: Sarcoplasmic reticulum. AP: action potential.
Not shown: Chloride-Kanal, Store operated Ca channel (TRPC1), Kalikum-Kanal K V , Phospholamban, α2-Receptor and others.
function
Smooth muscles can maintain a long-lasting tone ( tonic continuous contraction ) due to their structure and the processes described during contraction . Both the peristalsis in the stomach , intestines and urinary tract and the regulation of blood pressure in the inner walls of the arteries are based on the action of smooth muscles. During childbirth it enables rhythmic contraction ( phasic-rhythmic contraction ) of the uterus ( labor ).
Matrix production
Smooth muscle cells are used to synthesize collagen and other components of the extracellular matrix , such as B. proteoglycans , elastin and laminin .
pathology
Smooth muscle cell neoplasms are called leiomyomas . They are mainly found in the uterus and gastrointestinal tract . If the tumor becomes malignant, it is called leiomyosarcoma .
See also
Individual evidence
- ↑ Renate Lüllmann-Rauch: Pocket textbook histology . 5th edition. Thieme, Stuttgart 2015, ISBN 978-3-13-129245-2 , p. 238 and 260 .
- ↑ a b c d Renate Lüllmann-Rauch: Pocket textbook histology . 5th edition. Thieme, Stuttgart 2015, ISBN 978-3-13-129245-2 , p. 264 .
- ↑ Jan C. Behrends et al .: Dual series: Physiology . 3. Edition. Thieme, Stuttgart 2016, ISBN 978-3-13-138413-3 , p. 74 and 76 .
- ↑ a b Renate Lüllmann-Rauch: Pocket textbook histology . 5th edition. Thieme, Stuttgart 2015, ISBN 978-3-13-129245-2 , p. 260 .
- ^ A b Ernst Mutschler: Anatomy, Physiology, Pathophysiology of Humans. Wissenschaftliche Verlagsgesellschaft, Stuttgart 2007, ISBN 978-3-8047-2342-9 , 6th edition.
- ↑ a b c Renate Lüllmann-Rauch: Pocket textbook histology . 5th edition. Thieme, Stuttgart 2015, ISBN 978-3-13-129245-2 , p. 261 .
- ↑ a b Renate Lüllmann-Rauch: Pocket textbook histology . 5th edition. Thieme, Stuttgart 2015, ISBN 978-3-13-129245-2 , p. 262 .
- ↑ Renate Lüllmann-Rauch: Pocket textbook histology . 5th edition. Thieme, Stuttgart 2015, ISBN 978-3-13-129245-2 , p. 262 and 263 .
- ↑ a b c d e f g h Jan C. Behrends et al .: Dual series: Physiology . 3. Edition. Thieme, Stuttgart 2016, ISBN 978-3-13-138413-3 , p. 76 .
- ↑ a b c Renate Lüllmann-Rauch: Pocket textbook histology . 5th edition. Thieme, Stuttgart 2015, ISBN 978-3-13-129245-2 , p. 263 .
- ↑ a b Jan C. Behrends et al .: Dual series: Physiology . 3. Edition. Thieme, Stuttgart 2016, ISBN 978-3-13-138413-3 , p. 75 .
- ↑ a b c d Jan C. Behrends et al .: Dual series: Physiology . 3. Edition. Thieme, Stuttgart 2016, ISBN 978-3-13-138413-3 , p. 74 .
- ↑ Renate Lüllmann-Rauch: Pocket textbook histology . 5th edition. Thieme, Stuttgart 2015, ISBN 978-3-13-129245-2 , p. 264 and 265 .
- ↑ Jan C. Behrends et al .: Dual series: Physiology . 3. Edition. Thieme, Stuttgart 2016, ISBN 978-3-13-138413-3 , p. 569 .
- ↑ Jan C. Behrends et al .: Dual series: Physiology . 3. Edition. Thieme, Stuttgart 2016, ISBN 978-3-13-138413-3 , p. 560 to 565, as well as 570 and 572 .
- ↑ Jan C. Behrends et al .: Dual series: Physiology . 3. Edition. Thieme, Stuttgart 2016, ISBN 978-3-13-138413-3 , p. 564, 572 and 573 .