electron microscope
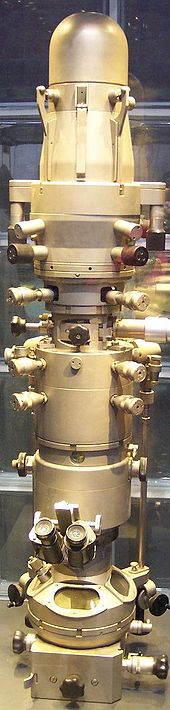
An electron microscope (formerly also an over microscope ) is a microscope that can depict the inside or the surface of an object with electrons . As with classic light microscopes , the resolution depends on the wavelength used. Since fast electrons have matter waves of a much shorter wavelength than visible light, a significantly higher resolution (currently about 0.1 nm ) can be achieved with an electron microscope than with a light microscope (about 200 nm). While the resolution of light microscopes actually reaches the physical limit caused by diffraction , in electron microscopes the aberrations of the electron-optical components worsen the usable resolution by around one and a half orders of magnitude compared to the theoretically possible resolution, which is around 0.0037 nm for 100 keV electron energy.
There are different types of electron microscopes that produce an image of the object in different ways:
- In transmission electron microscopes , electron-optical lenses are used which, by means of magnetic or electric fields, deflect the electron trajectories similar to light when it passes through light-optical converging lenses. This results in an analogy between traditional transmitted light microscopes and transmission electron microscopes up to and including the beam path.
- Secondary electron microscopes take a different approach to image generation, similar to confocal light microscopes .
construction
The main components of an electron microscope are:
- The electron gun that generates the free electrons in an electron source and accelerates them in the direction of an anode located in a ring around the beam axis . Electrically, the anode is at ground potential, the cathode at a negative high voltage, which, depending on the microscope, is between a few kilovolts and 3 megavolts. This voltage between cathode and anode determines the energy of the electrons.
- Electron lenses that can deflect the trajectories of electrons. Magnetic lenses are mostly used, in some cases electrostatic ones in the electron gun. Electron lenses have the same function as glass lenses in a light microscope. While the focal length of glass lenses is fixed, it can be regulated with electron lenses. Therefore, in contrast to a light microscope, an electron microscope does not contain any exchangeable or displaceable lenses (systems) like the objectives or the eyepiece of a light microscope. As with the light microscope, apertures are also used in addition to lenses.
- The vacuum system , which ensures that the electron source can work more efficiently and the electrons are not hindered on their way by colliding with gas molecules.
- The object holder, which must guarantee a stable position of the object. In addition, manipulation options are often desired, of which different combinations are realized depending on the type of object holder: displacement, rotation, tilting, heating, cooling and others.
- Detectors that register the electrons themselves or secondary signals.
- The microscope column forms the framework for all electron-optical components, usually shields magnetically in order to weaken the influences of external magnetic fields on the measurements, and seals the vacuum maintained inside.
species
Working principles and geometries
Electron microscopes can be divided according to two basic aspects.
- The first is the type of imaging:
- Scanning electron microscopes (SEM or, for engl. Scanning electron microscope , SEM) generate a electron-optical electromagnetic and electrostatic lens system has a fine electron beam on the object, which is line-wise performed on the rectangular to be examined object region ( "rastered"). The image is created through the synchronous registration of a signal triggered or influenced by the electron beam.
- Still image microscopes irradiate an object area with a fixed, wide electron beam. The image is generated here by using part of the electrons emanating from the object for image generation by means of an electron-optical system. It is important here that the electrons in the object - unlike light - are scattered in a highly inelastic manner and lose energy. The electron-optical imaging systems, however, show strong chromatic aberrations, so the inelastically scattered electrons disturb the optically generated image. In general, the idle mode can only be used if, after the interaction of the electron beam with the object, there are enough electrons with a sufficiently narrow energy distribution available and at the same time not too many electrons with different energies occur. This is naturally the case for sufficiently thin objects. The object thickness that can be examined can be increased if electrons of a suitable energy range are selected for imaging by means of electron energy filters.
- The second classification option relates to the geometry of the arrangement.
- In transmission is carried out by the fast electron beam may be used after passing through the object of imaging, with only very small scattering angles are included in the rule. Transmission electron microscope (TEM) usually work after the rest image method, occasionally the scanning method is applied here ( scanning transmission electron microscope (STEM) of English " scanning transmission electron microscopy / microscope "). The examined object areas must be very thin (one speaks of electron transparency, for acceleration voltages or electron energies that are common today, a maximum of a few 100 nm for very coarse resolution, typically less than 100 nm, for high resolution a maximum of a few 10 nm).
- If signals other than the transmitted electrons are mainly used to generate images, there is no fixed name for this. In the following table, which clarifies the given classification, o. B. d. A. Backscatter (this is not the physical term). If one wants to examine compact objects, this is the only possibility. The signals used are mostly secondary electrons, more rarely backscattered electrons. As a rule, this can only be done using the grid method (see above). An exception is the grazing incidence of electrons on almost flat solid surfaces, here a sufficient proportion of electrons is elastically reflected so that one can work in the idle image mode.
The combination of the above classifications provides the following matrix (by far the most common types are in bold):
Still image EM | Raster EM | |
---|---|---|
transmission | TEM | STEM |
Backscatter | Reflection microscope | REM (English SEM) |
The most common electron microscopes according to the number of devices installed are the SEM / SEM, followed by the TEM. STEM is even less common, although the STEM mode is often possible as an operating mode, particularly in the TEM devices developed since the mid-1990s, and pure STEM devices ( dedicated STEM ) are extremely rare. Reflection microscopes can only be found as laboratory structures in some institutes, but are not commercially available. Reflection microscopy can also be practiced in a normal TEM if the object surface can be placed almost parallel to the electron beam. Reflection microscopy, i.e. H. Electron-optical imaging of surfaces is used, for example, in short-term experiments in which the electron beam is only available for very short times; the short period of time would not be sufficient to scan the image field with an electron beam in the same way as with the SEM.
There is also the field electron microscope (also known as field emission microscope), which works without imaging optics, and in which the object itself forms the cathode from which the electrons emerge.
Some more special types of electron microscopes
Apart from the basic distinction, there are also a large number of designations that are more or less systematic and are mostly based on the area of application, additional technologies installed or certain special features of the respective devices. Some of them are given below:
- Analytical transmission electron microscope (ANTEM, equipped with EELS and EDS spectrometers, often also operable in STEM mode)
- energy-filtered transmission electron microscope (EFTEM, with imaging in-column or post-column EELS spectrometer)
- Environmental Scanning Electron Microscope (ESEM, especially for investigating atmospheric environments.)
- aberration corrected TEM, STEM and SEM
- Reflection electron microscope (a TEM in which the object can be tilted so that its surface is imaged under grazing electron incidence)
More detailed explanations of important basic types
Scanning electron microscope

With the scanning electron microscope (SEM; or English scanning electron microscope , SEM) a thin electron beam is scanned over the usually massive object. Electrons emanating from the object or backscattered, or other signals, are detected synchronously, the detected current determines the intensity value of the assigned image point (currently irradiated by the electron beam). Most of the time, the data is displayed immediately on monitors so that the image structure can be followed in real time. With old SEMs without a computer connection, a cathode ray tube was controlled directly with the signal intensity, and the image written on the fluorescent screen of this tube was then photographed with a camera with a correspondingly long shutter opening time to save the image.
The most important signals used in the SEM to map the object surface are secondary electrons (SE) and backscattered electrons (BE or BSE from back scattered electrons ). The cathodoluminescence (KL) signal (or English cathodoluminescence , CL) is of secondary importance and is only used in special studies.
The SE are low-energy electrons that are released by primary electron bombardment. This enables a very high resolution. The SE are accelerated by a suction voltage in the direction of the detector and there generate a number of electrical pulses corresponding to their quantity. A different image is generated depending on the position of the detector in the object chamber. The standard SE detector is attached to the side above the object and provides a very natural, three-dimensional image because the side facing the detector is brighter than the side facing away. In the past, an SEM that only worked in this mode was called a secondary electron microscope . Another SE detector present in modern SEMs is the so-called "Inlens" detector, which is attached in a ring above the object inside the column. Due to the very short working distance, it enables very high-resolution images (a few nanometers) with low acceleration voltages of the primary beam (a few hundred volts).
The BE or BSE are electrons from the primary beam that are elastically scattered at the atomic nuclei that are hit at or up to a few tens of nanometers below the object surface. The energy of the electrons is in the range of the irradiated primary electrons, the image resolution is in the micrometer range depending on the primary energy. The BSE detector is usually placed as a 4-quadrant semiconductor detector directly above the object. Depending on the wiring of the semiconductor crystals, different topography contrasts are obtained, with deep-lying areas of the object appearing dark. The property that heavy elements reflect the electrons more strongly than light ones is used with the so-called Z contrast (Z = atomic number of the elements). The brightness of the image area allows conclusions to be drawn about the chemical nature of the object surface.
Cathodoluminescence (KL) is the luminescence of the object surface triggered by electron bombardment. The KL signal, i.e. the light emitted by the object, is led out of the object chamber via special mirrors and light guides, spectrally broken down by means of a monochromator and detected by a photomultiplier or a CCD detector.
Another investigation method on the SEM (but also on the transmission electron microscope), which is currently gaining in importance, but which does not depict the object surface, uses electron backscatter diffraction . With their help one can determine the crystallographic orientation of crystals on the object surface. This is of great importance , for example, for the characterization of material properties in materials science and geology . For this purpose, the electrons reflected from the crystal surfaces of the object are projected onto a detector screen and the resulting Kikuchi lines are analyzed with the aid of a computer and assigned to crystallographic directions.
The electron microprobe is a special scanning electron microscope that is optimized to carry out chemical analyzes on surfaces in the micrometer range. This is where the wavelength dispersive (WDX) or the energy dispersive (EDX) X-ray analysis are used.
An ESEM ( environmental scanning electron microscope ) makes it possible to work with a relatively high gas pressure (a few dozen millibars ) close to the object. This makes it possible to examine moist objects (e.g. living cells or growing crystals).
Transmission electron microscope

In the transmission electron microscope (TEM), the electrons radiate through the object, which for this purpose must be correspondingly thin. Depending on the atomic number of the atoms that make up the object material, the level of the accelerating voltage and the desired resolution, the suitable object thickness can range from a few nanometers to a few micrometers. The higher the atomic number and the lower the accelerating voltage , the thinner the object must be.
By changing the projective lens system, instead of the intermediate image, the focal plane (focal plane) of the objective lens can also be enlarged (see illustration). An electron diffraction pattern is obtained , with the help of which the crystal structure of the object can be determined.
The transmission electron microscope can be useful extended with various analytical methods are particularly common energy dispersive X-ray analysis ( energy-dispersive X-ray analysis , EDX, and energy dispersive X-ray spectroscopy, EDS, called) and electron energy loss spectroscopy (English electron energy loss spectroscopy , EELS). Both methods can be used to determine the concentration and distribution of chemical elements in the object, the small achievable diameters of the electron beam in principle also allowing the investigation of very small object areas. When using these methods, one often speaks of analytical transmission electron microscopy.
Energy- filtered transmission electron microscopy (EFTEM) is a further development of the electron energy loss spectroscopy method in TEM , in which mostly images of inelastically scattered electrons of specific, characteristic energies are recorded. This means that the distribution of chemical elements in the image field can often be determined very quickly and effectively. Similarly, energy-filtered electron diffraction images can also be recorded.
If the primary electron beam finely converged scanned over the object, the transmitted electrons are detected and the respective associated beam position on the object, so is referred to this method as a scanning transmission electron microscopy (STEM English scanning transmission electron microscope ).
Optical aberrations in electron microscopes
Real optical lens elements, both in light and electron optics, show deviations from the ideal behavior. In beam optics, for example, parallel rays that run through an ideal converging lens are deflected in such a way that they all intersect at the focal point after the lens. This is not the case with a real lens. In the wave optics image, a group of parallel light rays is represented by a plane wave. The effect of the converging lens transforms this plane wave into an incoming spherical wave. The aberrations of real converging lenses are expressed in this model by a relative phase shift of various components, so that the waveform no longer corresponds to that of an exact spherical wave.
While lens systems in light optics can be designed to be virtually aberration-free at least for certain areas of the light wavelengths by suitable choice of material and shape, this is not possible in the lens elements of electron optics. The lenses have a rotationally symmetrical magnetic dipole field (the axis of symmetry is the optical axis of the lens). The shape of the field is therefore basically predetermined, and O. Scherzer showed as early as 1936 that such lenses inevitably have strong spherical aberrations. With the help of combinations of magnetic multipole elements, which are also brought into the beam path, such aberrations can be corrected to a certain degree ( C s corrector , stigmator ); this has a certain similarity to the correction of optical errors in high-quality camera lenses ( spherical aberration , C S ) or human astigmatism through cylindrical glasses , see also imaging errors . However, since the aberrations depend on the current state of the lens (temperature distribution, electron-optical adjustment and other parameters play a major role here), the aberrations must be measured promptly and the correction elements controlled accordingly.
A measurement method for the optical errors (aberrations) of a transmission electron microscope (TEM) is the Zemlin tableau . In the TEM, images of foils made of amorphous material (mostly amorphous carbon) are recorded with different beam tilts. The power spectra of these images are arranged in a tableau according to the azimuth of the beam tilt. With the help of this table all paraxial aberrations can be measured. The Zemlin panel is used to precisely adjust the electron microscope and correct optical errors.
At first, aberration correction was only used to improve the spatial signal resolution (the information limit) of TEMs and STEMs (before the correction was introduced in the mid-1990s at around 0.11-0.15 nm). The resolving power of SEMs is usually not given by the smallest possible electron beam diameter due to their mode of operation, since the electron beam is strongly scattered by the object itself. However, the use of aberration correctors in SEMs allows higher beam currents (i.e. faster image recording) on the one hand and the compensation of reduced electron energies on the other, which initially leads to a larger beam diameter due to the increase in wavelength.
Object preparation
For the investigation in the normal SEM the sample should be conductive or coated with a conductive layer, as long as special techniques are not used, see scanning electron microscope .
For transmission electron microscopy (both CTEM and STEM), the objects must be brought to a maximum thickness of usually 10–100 nm (in special cases about 1 µm is sufficient) using suitable methods, see transmission electron microscope .
The surface morphology of massive objects can be examined with the transmission electron microscope by making a carbon imprint of this object that can be radiated through and is brought into the electron microscope with the help of a carrier network. The best way to do this is to use thin layers of carbon, which is evaporated from an arc in a high vacuum at a certain angle of inclination (e.g. 45 degrees) and then detached from the surface to be examined.
disadvantage
The time-consuming preparation of the objects can lead to artifacts - structures that were only created by the preparation and have nothing to do with the actual object - which makes it difficult to evaluate the images. In addition, the material properties in the SEM can deviate from those of compact objects due to the disproportionate share of near-surface areas in the analyte volume . Another problem is the damage to the objects by the electron beam, for example by heating or pushing away entire atoms after colliding with the fast electrons, but also by injection of foreign atoms from the vacuum into the sample. The vacuum prevailing inside the microscope, the drying and fixation required to produce a specimen, as well as the indispensable extremely fine cutting of the specimen make it impossible (except with the ESEM) to microscope a living object.
Another disadvantage is the very high acquisition and maintenance costs for electron microscopes, which often do not allow private companies to operate their own devices. This is why electron microscopes are mainly found in research institutes and service companies.
history

The first lens based on magnetic forces was developed by Hans Busch in 1926 . Ernst Ruska and Max Knoll built a TEM as the first electron microscope - also known as an over microscope at the time - in 1931 , although initially no electron-transparent objects but small metal grids were imaged for testing purposes. For this work Ruska received the 1986 Nobel Prize in Physics . He also developed the first commercial electron microscope at Siemens in 1938.
At about the same time as Ruska and Knoll, Reinhold Rüdenberg built an electrostatic electron microscope, for which he received a patent in 1931.
Contrasting biological objects with osmic acid was proposed by Ladislaus Marton in 1934. The first STEM was built in 1937 by Manfred von Ardenne .
The first Soviet electron microscope was built by Wiktor Werzner in 1940 .
While in the early years the elucidation of pathogens ( viruses ) that were invisible under the light microscope was an important driving force behind the development of the electron microscope, interest later expanded to include materials science in particular, after Robert D. Heidenreich succeeded in preparing thin radiolucent metal foils in 1949.
In the 1960s, TEM was developed with ever higher acceleration voltages (up to 3 MV, around 1965 in Toulouse , 1970 in Osaka ), primarily to be able to penetrate thick objects. Atomic resolution was also achieved for the first time in this decade.
At the end of the 1960s, Albert Crewe introduced the field emitter for STEM and thus made this technology important.
The ESEM was developed in the late 1980s. Schottky field emitters have been used in TEM since the late 1980s . FESEMs with Schottky field emitters have been in use since the early 1990s.
It is also worth mentioning the increasing use of computers since the 1990s. Complicated lens systems, for example, can be adjusted automatically by analyzing the recordings of a CCD camera, which significantly relieves the user of the microscope. The use of computers to compensate for aberrations of the electron-optical lenses with magnetic multipole lenses is indispensable, a technique that has become more and more important in recent years in the SEM, TEM and STEM fields.
At the beginning of 2008 a new transmission electron microscope with aberration correction, called “TEAM”, was announced. It has a resolution of 0.05 nm.
In December 2008 Forschungszentrum Jülich announced the construction of a 15 million euro laboratory with an electron microscope at the Ernst Ruska Center for Microscopy and Spectroscopy. With a resolution of 0.05 nm as well, it will be one of the highest resolution microscopes in the world.
On the occasion of the award of the Kavli Prize for Nanotechnology 2020 to Maximilian Haider et al. was called a record resolution of 43 picometers - less than the atomic diameter of hydrogen. Haider heads the company CEOS (Corrected Electron Optical Systems), founded in 1996, which manufactures imaging elements for electron microscopes. Together with Harald Rose (physicist) and Knut Urban, Haider received the Wolf Prize in Physics in 2011 for improving the resolution of electron microscopes and all three of them received the Kavli Prize in 2020 with Ondrej Krivanek for achievements in the field of electron microscopy. Krivanek developed aberration correctors (up to 3rd order), methods of electron energy loss spectroscopy (EELS), demonstrated sub-Angstrom electron microscopy and the coupling with vibration spectroscopy, for example in biology and analytical chemistry.
In addition to Ruska , the Nobel Prize for electron microscopy was also awarded to Jacques Dubochet , Richard Henderson and Joachim Frank ( cryo-electron microscopy , Nobel Prize for Chemistry 2017).
Trivia
In 1970 it was estimated that less than a cubic millimeter of material had been explored using an electron microscope, due to the thin layers and the high magnification.
See also
- Scanning tunnel microscope (STM or RTM)
- Field ion microscope (FIM)
- Atomic Force Microscope (AFM)
- Photoemission Electron Microscopy (PEEM)
- Boersch effect
literature
- Stanley L. Flegler, John W. Heckman Jr., Karen L. Klomparens: Electron microscopy: fundamentals, methods, applications . Spectrum, Heidelberg / Berlin / Oxford 1995, ISBN 3-86025-341-7 .
- Ludwig Reimer, Gerhard Pfefferkorn: Scanning Electron Microscopy . 2nd Edition. Springer, Berlin 1999, ISBN 3-540-08154-2 .
- David B. Williams and C. Barry Carter: Transmission Electron Microscopy. A Textbook for Material Sciences . Plenum Press, New York / London 1996, ISBN 0-306-45247-2 .
Web links
- The electron microscope - the whole story
- The Electron Microscopy Site ETH Zurich website: very good graphics and images that illustrate various processes.
- Electron microscopic atlas of cells, organs and tissues of mammals
Individual evidence
- ↑ O. Scherzer: About some defects in electron lenses. In: Journal of Physics. 101, No. 9-10, 1936, pp. 593-603.
- ↑ F. Zemlin, K. Weiss, P. Schiske, W. Kunath, K. -H. Herrmann: Coma-free alignment of high resolution electron microscopes with the aid of optical diffractograms . In: Ultramicroscopy . tape 3 , 1978, p. 49-60 , doi : 10.1016 / S0304-3991 (78) 80006-0 .
- ↑ Heinz Müller: Preparation of technical-physical objects for the electron microscopic examination . Academic publishing company Geest & Portig K.-G., Leipzig 1962.
- ↑ Bodo v. Borries, Ernst Ruska: The super microscope as a continuation of the light microscope. In: negotiations d. Ges. German natural scientists and doctors, 95th meeting in Stuttgart from 18.-21. September 1938. pp. 72–77 ( online in the Ernst Ruska archive ).
- ↑ Ernst Ruska: The electron microscope. In: Journal of Physics . 78, 1932, pp. 318–339 ( Online in the Ernst Ruska Archive ( Memento of the original from January 18, 2012 in the Internet Archive ) Info: The archive link has been inserted automatically and has not yet been checked. Please check the original and archive link according to the instructions and remove then this note. ).
- ↑ TEAM meets 0.5 Å milestone. In: The TEAM Project. Archived from the original on March 10, 2011 ; Retrieved July 11, 2012 .
- ↑ The most powerful microscope in the world. ( Memento of the original from December 21, 2015 in the Internet Archive ) Info: The archive link was inserted automatically and has not yet been checked. Please check the original and archive link according to the instructions and then remove this notice. weltderphysik.de, January 24, 2008.
- ^ TEAM Project Achieves Microscopy Breakthrough. (No longer available online.) FEI Company, archived from the original on December 11, 2007 ; accessed on March 25, 2008 (English). Info: The archive link was inserted automatically and has not yet been checked. Please check the original and archive link according to the instructions and then remove this notice.
- ↑ Wolfgang Müller: Most powerful microscope in the world. In: focus.de. FOCUS Online, accessed on February 20, 2016 .
- ↑ The world's most powerful microscope comes to Jülich. In: fz-juelich.de. Forschungszentrum Jülich GmbH, accessed on February 20, 2016 .
- ↑ Austrian physicist Haider receives Kavli Prize orf.at, May 27, 2020, accessed May 27, 2020.
- ^ Limas source number 473. Manfred v. Heimendahl: Introduction to Electron Microscopy. Friedrich Vieweg & Sohn, Braunschweig 1970, p. 1 ff. ( Memento of the original from November 29, 2014 in the Internet Archive ) Info: The archive link was automatically inserted and not yet checked. Please check the original and archive link according to the instructions and then remove this notice. Korpora.org, accessed November 15, 2014.
Remarks
- ↑ The term over microscope had already been used for other devices, e.g. B. in 1903, see Das Uebermikoskop. In: Ostdeutsche Rundschau. Viennese weekly for politics, economics, art and literature / Ostdeutsche Rundschau. Deutsches Tagblatt , September 26, 1903, p. 8 (online at ANNO ).