Confocal microscope

A confocal microscope (from confocal or confocal , having the same focus ) is a special light microscope . In contrast to conventional light microscopy, not the entire specimen is illuminated, but only a fraction of it at any point in time, in many cases only a small light spot. This lighting is rasterized piece by piece over the specimen . A complete picture is never created in the microscope. The light intensities of the reflected light or light emitted by fluorescence are consequently measured one after the other at all locations of the area to be imaged, so that a subsequent construction of the image is possible. In the beam path of the detected light there is a pinhole aperture that lets light through from the area in focus and blocks light from other planes. As a result, only light from a small volume around the focal point reaches the detector, so that optical sectional images with high contrast are generated, which almost only contain light from a narrow layer around the respective focal plane .
Today's confocal microscopes are available in different designs. Are widespread point scanners, in which a focused laser beam scans the specimen (confocal laser scanning microscope , engl. Confocal laser scanning microscope , CLSM, and LSCM according engl. To scan : rasterize). With line scanners, on the other hand, a whole line of images is created at once, so that a higher speed can be achieved. A third variant uses a Nipkow disc on which several perforated diaphragms are arranged in a spiral. As the disk rotates , each pinhole scans a circular curve of the specimen. This variant either uses white light for incident light illumination, which leads to reflection in the specimen. Or fluorescence is excited, as is also possible with the other types of construction. Then they belong to the fluorescence microscope .
The first confocal microscopes were built in the middle of the 20th century. However, it was not until the 1980s that new technical possibilities, including lasers and computer systems , allowed further developments that led to greater dissemination.
The confocal principle
A point light source is imaged into the specimen in a confocal microscope. From the spot illuminated in this way, light is focused through the lens onto a pinhole before it reaches the detector. The point in the middle of the pinhole and the illumination point in the specimen are confocal to one another, i.e. they are in focus at the same time.
Confocal microscopy can in principle be carried out with transmitted light or with incident light . Today's confocal microscopes are generally reflected light microscopes, they use the objective for illumination and detection. In some building types there is not just one, but several lighting points that are projected simultaneously onto different points on the specimen. For the sake of simplicity, the principle is explained here using the example of a single point of illumination that is generated by incident light.
Beam path from the light source to the specimen
The light coming from the light source (green in the schematic drawing) is first focused into the excitation pinhole to generate a point light source. Also in German usage, this and the second pinhole are often referred to with the English expression pinhole (literally: needle hole). In more recent confocal laser scanning microscopes, the illumination lasers are coupled with glass fibers and the excitation pinhole can then be replaced by these, since the small light-guiding fiber core has similar optically restricting properties.
The lighting is passed on to a beam splitter . With white light microscopy, a semitransparent mirror is used that reflects a sufficient proportion of the light on the specimen. If fluorescence is to be detected in the specimen, a dichroic mirror is used that reflects the excitation light but lets the fluorescent light through. Finally, a reduced image of the excitation pinhole is projected through the objective in the specimen. Due to the diffraction , a diffraction disk (more precisely: a point spreading function ) and not an actual point is created at the focal point.
Beam path from the specimen to the detection pinhole
The light to be detected emanates from the illuminated point in the specimen (red in the schematic drawing). This can be reflected light or fluorescence. The part picked up by the objective passes through the beam splitter and is reunited in the intermediate image plane in one point (a diffraction disk). In the case of modern objectives with an infinite tube length , a tube lens is also required for this. The detection pinhole is centered around this point in the intermediate image plane. It is typically just big enough that its edge runs in the first minimum of the diffraction disk. The actual diameter therefore depends on the numerical aperture and the magnification of the objective used. A further enlargement can also be made up to the aperture plate level in order to increase the diameter of the diffraction disk and thus the diameter of the aperture plate. Larger panels are easier to manufacture.
In most specimens, light is not only emitted from the actually illuminated point in the specimen, but also from areas above or below (pink in the diagram). For example, fluorescent dyes are also excited in planes above and below the plane of focus. The light coming from these points is, however, not combined into one point in the intermediate image plane, but in planes in front of or behind it, so that the light rays from these points are present as beam cones in the intermediate image plane. The majority of these beam cones are therefore blocked by the perforated diaphragm so that very little light reaches the detector that was not emitted from the focal point in the specimen.
Optical information that does not come from the focal point of the specimen is thus suppressed twice: Firstly, it is not “queried” because the illumination intensity outside the focus is weak, and secondly, light from outside the focal point is almost completely blocked at the pinhole. This results in a significant improvement in contrast and a slightly better resolution .
The so-called “focal point”, from which the light contributes to the creation of the image, is a three-dimensional volume. The exact shape of this volume is a point spread function (Engl. Point spread function, abbreviated PSF), respectively. The smaller the volume, the better the resolution of the microscope.
Since only reaches from a small volume of the preparation light to the detector, it is possible for the image forming necessary to move this volume above the specimen, so scan the sample. Even if several focus points are used, they must be moved over the specimen. In the microscope itself, a complete image of the specimen is never created; this is only then generated in the computer or, in the case of several points, on the film or the chip of a camera.
The confocal laser scanning microscope - scanning with a focused laser beam

In biomedical research, confocal laser scanning microscopes (abbreviation CLSM, in English: confocal laser scanning microscope , more rarely LSCM, also German: laser scanning microscope ) are widespread, in which a laser beam focused by the lens scans an object point by point . Usually fluorescence is detected by special markers; it is then a form of reflected light fluorescence microscopy . Lasers are used because not enough light from other light sources for intensive fluorescence excitation can be concentrated on one point. Confocal reflection microscopy can also be performed with such devices.
The optical elements additionally required for confocal microscopy are housed in a box in commercial devices that is flanged to a high-quality microscope. The laser light is directed into this scanner box and reflected from there into the microscope in order to reach the specimen through the objective. The signal generated in the specimen goes the opposite way back to the box, where the signal intensity is measured behind the pinhole. The recording process is controlled by a computer and the images are displayed on the computer monitor.
From laser to preparation
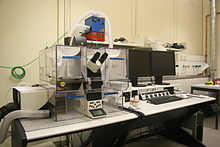
Typically, these devices have multiple excitation lasers , for example an argon laser that emits multiple wavelengths (458, 488, 514 nm, and others) and helium-neon lasers that emit light at 543 or 633 nm. The light of different colored lasers can be superimposed with dichroic beam splitters . In many current devices, the intensity of the respective wavelengths is modulated via an acousto-optical modulator (also: acousto-optical tunable filter, AOTF).
In newer devices, the laser light is often guided to the scanner box via glass fibers . Since the small light-guiding core of a glass fiber looks similar to a pinhole, the excitation pinhole can be omitted. The light now falls on the dichroic beam splitter , which directs it to the first scanning mirror. When using several excitation wavelengths, double or triple beam splitters can be used, which reflect two or three wavelengths towards the preparation, but allow the respective fluorescent light to pass through. In some devices, this function is performed by another acousto-optical modulator, which is then referred to as an acousto-optical beam splitter (AOBS).
The movement of the scanning mirror determines how fast and in which area the excitation point (more precisely: the diffraction-limited excitation volume) scans over the specimen. The scanning speed is specified in image lines per second, i.e. in Hertz (Hz). Typical speeds are between 200 and 2000 Hz. The size and position of the scanned area are determined by a precisely controlled deflection of the mirror. The excitation point is continuously moved over the preparation within an image line and the number of image points ( pixels ) per line is determined by defining the so-called pixel dwell time ( pixel dwell time , time until the signal is assigned to the next pixel ). Together with the number of image lines, this gives the total number of image points, for example 512 × 512 pixels. Variable control of the scanning mirrors therefore makes it possible to record images that have been enlarged to different degrees with the same lens. This is an important difference to camera-based systems.
With additional lenses and mirrors, the excitation light is finally guided through the objective onto the specimen, where fluorescence excitation or reflection occurs.
From preparation to detector

The fluorescent light takes the same path back via the scanning mirror, passes the dichroic beam splitter and, in accordance with the confocal principle described above, reaches the pinhole in an intermediate image plane and finally to the detectors. Alternatively or additionally, reflected light can also be captured via this beam path. In addition to the resolving power of the objective used (more precisely: its numerical aperture ) and the wavelength of the respective light, the diameter of the pinhole determines the depth of field and thus the “thickness” of the optical section. If the diameter of the pinhole is in the first minimum of the diffraction disk (1 Airy unit), most of the light from other planes is blocked and most of the actual signal passes through. If the diaphragm is opened more, more light comes through from higher and lower preparation levels, so that more blurred parts contribute to the image (see illustrations). If the shutter is closed more strongly than an Airy unit, there is a sharp drop in brightness, which also makes a clear picture more difficult.
In order to be able to record several fluorescent colors or reflected and fluorescent light in parallel confocally, the light is spectrally separated in front of the detector. Theoretically, the size of the detection pinhole would have to be adjusted for each wavelength, since the diameter of the diffraction disk is linearly dependent on the wavelength. In fact, in some earlier devices (for example in the Zeiss LSM 410), the spectral separation happened first and each color area then had its own pinhole. However, for practical reasons, today's commercial devices only use one pinhole for all colors. The spectral separation only takes place behind this, for example with dichroic beam splitters that direct different color components onto different detectors.

Photomultipliers (PMTs) are mostly used as detectors in commercial devices and sometimes also avalanche photodiodes (APDs) are used in special applications . New hybrid photodetectors (HPDs) combine properties of PMTs and APDs. Like many normal PMTs, they are manufactured by Hamamatsu Photonics and installed by various microscope suppliers.
Some devices have an additional detector in the beam path behind the specimen, which collects laser light that passes through. In the computer, a kind of bright field image can be reconstructed from the measured values, in which light-absorbing or deflecting structures in the preparation are represented by dark areas. The light does not pass through a pinhole on the way to this detector, so that no confocal image is created.
Special features of the recording
In order to increase the signal-to-noise ratio and thus the image quality, the control software allows a picture to be recorded several times and the mean value to be calculated. This helps to reduce the influence of the Poisson noise of the captured photons and the statistical noise of the electronic components on the image, especially in the case of weakly fluorescent specimens , since noise is distributed differently with each exposure and is therefore less important for multiple exposures.
Today's commercial confocal laser scanning microscopes can shift the focal plane step by step by moving the objective or the specimen in order to generate optical serial sections (see film sequence). Such image series can be used as a basis for three-dimensional computer reconstructions (see figures).
Point scanning method without scanning mirror
Early confocal microscopes had an immobile beam path, instead the specimen was moved, as this was technically easier to achieve (see below, history ). Although this “stage scanning” approach is significantly slower due to the mass to be moved, it has the advantage that the illumination intensity is exactly the same for every point on the specimen. In the case of “beam scanning”, i.e. the movement of the laser beam over the non-moving specimen shown above, the illumination at the edge of the field of view is slightly less intense. For applications for which this is important and high speed is not required, stage scanning can therefore also be used today (as of 2013), for example fluorescence correlation spectroscopy (FCS). With FCS, the fluorescence intensity in the detected volume is measured over a longer period of time at one point, so that confocality is absolutely necessary, but no scanning device.
A third possibility to scan a plane of the specimen is to move the objective sideways. This option is rarely used. The first confocal microscope with laser used this approach (see below ), but current (2017) commercial devices are also available with this option.
Commercial devices
Confocal laser scanning microscopes with beam scanning for the life sciences are offered by all four major microscope manufacturers ( Carl Zeiss , Leica Microsystems , Nikon and Olympus ). Some of these devices combine confocal microscopy with other laser scanning microscopy applications such as multiphoton microscopy or STED microscopy . Bio-Rad , the first manufacturer of commercial confocal laser scanning microscopes, has meanwhile sold its microscope division to Zeiss, so that the brand no longer appears in the microscope market. There are also a number of manufacturers who offer confocal laser scanning microscopes for special applications. These include PicoQuant, HORIBA, ISS and WITec.
Special applications with confocal laser scanning microscopes
The special properties of the confocal laser scanning microscope allow other applications in addition to the production of optical cuts. In some cases, additional equipment is required for this. These techniques include fluorescence recovery after photobleaching (FRAP), fluorescence correlation spectroscopy (FCS), single molecule fluorescence spectroscopy , Förster resonance energy transfer (FRET), Raman spectroscopy, and fluorescence lifetime microscopy (FLIM).
Line scanner
Point scanners are relatively slow because each point in the specimen has to be scanned individually. This is not just a technical problem of the maximum speed that can be achieved: if the individual image points are scanned too quickly, insufficient fluorescent light is also captured to create an image with sufficient contrast . One way of avoiding this problem is to illuminate the specimen with a line (instead of a point) and guide the fluorescent light through a corresponding slit instead of through a pinhole. This results in a different ( anisotropic ) resolution in the x and y directions. In the direction of the scan line and the slit diaphragm (x), the resolution corresponds to a conventional microscope, the advantages of the confocal microscope only come into play perpendicular to it (y-direction). Before the development of spinning disk microscopes that are suitable for fluorescence microscopy, line scanners were by far the fastest way to create confocal images of weakly fluorescent specimens.
The main technical difficulty in the construction and operation of line scanners is the realization of a diffraction-limited, narrow excitation line with uniform brightness and a sufficiently narrow slit diaphragm as well as the alignment of the two exactly parallel to each other. The alignment not only requires movement in the x and y directions, as with the point scanner, but also rotation. If the two edges of the slit diaphragm are not completely even and parallel to one another, this can lead to streaks in the image, for example if dust accumulates on the edge of the diaphragm. In practice, these problems meant that both the excitation line and the slit diaphragm were considerably wider than they should theoretically be.
The line of fluorescent light can either be captured with a CCD line detector (with the LSM5 live and Duo devices from Zeiss), or the line is mapped onto a camera using a movable mirror so that an image is built up line by line on the camera chip (Meridian Insight, BioRad DVC 250). Due to the high scanning speed, this image can also be viewed with the eye through an eyepiece.
Instead of one line, the specimen can also be illuminated with several adjacent lines. The Zeiss CSM 700, which is intended for the surface examination of materials, uses a slit mask in the illumination beam path to create a striped pattern on the specimen. For detection, the focal plane is mapped onto the chip of a camera, the function of the slit diaphragm is digitally simulated by only reading out certain pixels. By moving the slit mask, the specimen is finally fully captured. Since white light is used for excitation, images can be recorded in real color. The contact-free examination of surfaces is made possible by only working with dry objectives.
Confocal microscopes with Nipkow discs - scanning with many focused light beams
Another possibility for fast confocal recording is the use of many pinhole diaphragms used in parallel on a Nipkow disk . The name is a bit misleading: The disc that Paul Nipkow developed in the 19th century for the transmission of television images contained a spiral with holes. In contrast, Nipkow disks used for confocal microscopy contain many spiral arms lying close together. The lighting hits the pane and partially passes through the perforated diaphragms. These are made smaller in the specimen in order to generate many focal points.
By rotating the disk, the focal points scan in arcs of a circle very quickly over the specimen, so that a complete image is created in a fraction of a second. This means that the image can also be recognized with the eye. A camera is used as a detector and the examined specimen section is scanned once during the minimum exposure time.
For fluorescence microscopy, microscopes with Nipkow disks were initially unsuitable, as the holes in the Nipkow disk let through less than one percent of the illumination. As a result, the excitation is too weak for most fluorescence-marked preparations. Although there are devices available for use in the life sciences, they are not widely used. It was only around the turn of the millennium that the strength of the stimulation was improved by new technical approaches.
Tandem scanner for white light reflection microscopy
In the 1960s, the Czechoslovak Mojmír Petráň developed the "Tandem Scanning Microscope" (TSM), which made confocal reflection microscopy possible. It is so called because the illuminating beam path goes through one side of the Nipkow disk and the imaging beam path goes through the opposite side. The perforated diaphragms on both sides therefore raster when the disc rotates in tandem. Each illumination pinhole corresponds to a detection pinhole in exactly the opposite position.
For the original version of the microscope, the Nipkow disk with a diameter of 8.5 cm was made from 20 micrometers thin copper foil , into which 26,400 holes with a diameter of around 90 micrometers and an average distance of 280 µm from hole center to hole center were etched. The holes were arranged in 80 Archimedean spirals . The disk rotated three times per second, the observation field was scanned 120 times per second. The source of illumination was a tungsten lamp or, for stronger illumination, an image of the sun.
The light that passes through the Nipkow disk (highlighted in red in the drawing) passes after several reflections to a partially transparent mirror (above the lens, 3), which reflects part of the lighting to the lens. The part of the lighting that passes through this mirror is lost. The objective images the pinhole diaphragms in the specimen. The light reflected at these points is picked up again by the lens and directed to the partially transparent mirror. This time only the part that passes through is of interest: it is directed through several reflections to the opposite side of the Nipkow disk. A sophisticated manufacturing technique ensures that both halves of the pane are optically identical, so that the light reflected in the illumination points from the focal plane can now pass through the pinhole diaphragms in accordance with the confocal principle.
Theoretically, lighting with white light gives a true color image. However, even very good glass optics can not completely avoid chromatic aberration . As a result, the lighting in the preparation for different colors is in slightly displaced levels.
In the first publication on Petráň's microscope, the imaging of spinal ganglia and brains was described. Tandem scanning microscopes are still used today, for example to examine the cornea of the eye .
Single-sided Nipkow disk microscopes for white light reflection microscopy
In contrast to the tandem scanner, the Nipkow disc is only irradiated on one side: the same pinhole diaphragms are used first for the lighting and then for the detection.
Coming from above, the lighting first hits a beam splitter, which directs part of the light to the Nipkow disk. The part that passes through here reaches the specimen through the objective, where a focal point is created corresponding to each pinhole. Reflected light falls back into the lens and continues through the same pinhole towards the beam splitter. There it is partially mirrored to the camera, the rest is lost.
It is important to avoid that light reflected from the Nipkow disc itself reaches the camera. This can be achieved by mirroring the Nipkow disk and tilting it a little. However, the pinhole diaphragms are then no longer in the optimal position. To compensate for this, they need to be made a little bigger. Alternatively, you can work with polarized light.
Such microscopes for reflection images are used in materials science to examine surfaces, for example in the semiconductor industry. Here they are more suitable than confocal laser scanning microscopes, since the coherent light of a laser when reflected on smooth surfaces leads to undesirable interference effects that are avoided when illuminated with non-coherent white light.
Powerful spinning disk microscopes for fluorescent specimens
A second disc with microlenses
The Japanese company Yokogawa was the first to develop a confocal Nipkow disk system that is suitable for fluorescence excitation, the Yokogawa spinning disk. Above the Nipkow disk there is a second disk that rotates synchronously and on which microlenses are applied. Each pinhole is therefore preceded by a microlens that focuses the light on the opening. In this way, over 60% is let through to the preparation. The dichroic beam splitter required for fluorescence microscopy is located between the two panes and decouples the fluorescence that comes back from the specimen to the side, towards the camera. As a result, the fluorescent light goes through the pinhole diaphragms, but not through the microlens disc, so that light losses are avoided here. In addition, scattered light that occurs in the excitation beam path when it strikes the microlens disc cannot reach the camera.
The good light output of the system with around 2000 confocal pinhole diaphragms used simultaneously enables real-time observations with a significantly better signal-to-noise ratio than typical confocal point scanners with a comparable number of images per second. Since an image point can not only be scanned once, but more frequently in succession, the maximum light point load in the preparation is lower than in typical point scanners, so that the fluorochromes in the preparation are less bleached . The disks rotate at 5 or 10 revolutions per second. Some published works have achieved frame rates of 15 frames per second. The redundant patterns on the disks mean that less than one disk revolution is required to capture a complete image. Due to the high frame rates, in contrast to the point scanner, it is also possible to look at the confocal image through the eyepiece with the eye.
Yokogawa itself does not offer microscopes. Instead, the component described is built into their own devices by other companies, such as Leica Microsystems, PerkinElmer and Zeiss. Lasers are used as the light source because they enable uniform and strong lighting. Therefore, this type of device is also a confocal laser scanning microscope. However, the name “spinning disk” microscopes has established itself.
A disc with concave mirrors
Till Photonics in Graefelfing developed an alternative approach for a good light yield for fluorescence excitation with Nipkow disks . In this “Andromeda” system, laser light is fed in via a so-called corner cube. The light initially goes straight through this, is reflected by the dichroic beam splitter and continues through a lens to the Nipkow disk. Each of the many pinhole diaphragms on the disk is surrounded by a hexagonal concave mirror . Only a small amount of light immediately passes through the pinhole diaphragms. The rest is reflected back and meets the corner cube after the dichroic beam splitter. From this side the corner cube looks like a flat mirror so that the light is directed back to the Nipkow disk. During this second pass, due to the effect of the concave mirror, most of the light passes through the pinhole diaphragms and hits the specimen. According to the confocal principle, the fluorescent light coming back from the specimen passes through the Nipkow disk to the dichroic beam splitter and then to the camera. One advantage of this system is that the dichroic beam splitters are in an infinite area of the beam path. As a result, their characteristics are less critical for the image quality.
Advantages and disadvantages of confocal Nipkow disk systems compared to point scanners
The simultaneous use of many pinhole diaphragms and thus many points of illumination means that systems based on Nipkow disks can scan a specimen faster than a point scanner. However, in a conventional Nipkow pane system, most of the lighting on the pane is lost. While the amount of light passing through is still sufficient with confocal white light microscopes, light-maintaining devices are required for fluorescence microscopy. However, systems of this type then allow living fluorescent cells to be picked up more gently, since the maximum light exposure of each point is lower (see above).
By using several perforated diaphragms lying next to each other in the Nipkow disk, it is possible that light from one point in the specimen can reach the camera through the wrong perforated diaphragm and thus limit the confocality of the image. This effect rarely occurs in material examinations of surfaces. However , this can become a problem with thicker biological specimens that are scattered . The confocal effect is then weakened with increasing depth of the focal plane.
Furthermore, with Nipkow disk systems, the observable region in the preparation is fixed. Zooming in or out, i.e. changing the size of the scanned region as with the point scanner, is not possible. This is also unproblematic in material examinations, since the samples do not fade and thus a scaled-down region is not an advantage. A higher magnification can also be achieved here by switching between the dry lenses or by using a magnifying lens directly in front of the camera. On the other hand, spinning disk fluorescence microscopes used for live cell observations are usually of the inverse design and immersion objectives are used, since these capture a larger proportion of the fluorescence due to the higher numerical aperture . Since the immersion liquid has to be removed and reapplied when changing lenses, changing the lens is more time-consuming. Additional zoom optics always lead to a slight loss of light due to reflections on the additional glass surfaces, which is why these are dispensed with in the case of weak fluorescence observations in favor of higher sensitivity.
Different objectives produce diffraction disks of different sizes, depending on the magnification and numerical aperture. The size of the pinhole diaphragms on a disk cannot be changed, so that the optimum size can only be achieved for a single objective or for a few objectives. In the case of point scanners, on the other hand, the size of the pinhole can be adapted to the objective and the excitation wavelength used.
One-sided Nipkow disk systems have the advantage that an adjustment of the excitation and emission pinhole diaphragms to one another is not necessary, as is the case with point scanners, since the same set of pinhole diaphragms is used for both purposes.
Resolving power, optical sections and positioning accuracy
resolution
As is generally the case with light microscopes, the resolution of confocal microscopes is limited by diffraction . A point light source is due to the diffraction of three-dimensional point spread function (engl. Point spread function , PSF) imaged. The section through the middle plane of the PSF is called the diffraction disk (see illustration). For practical reasons, in the confocal microscopy instead of the resolution ( Rayleigh criterion which) often half-width (ger .: full width half maximum , FWHM) of the PSF indicated, ie the width in which 50 percent of the maximum brightness are available. These values are generally somewhat lower than the actual resolution.
Due to the pinhole diaphragms in the illumination and detection beam path, the confocal resolution can be somewhat better than in conventional microscopes. The greatest possible theoretical improvement in resolution by the factor is only achieved when the detection pinhole is almost completely closed, so that no more light would then be captured and therefore no image would be created.
The resolution that can actually be achieved is therefore only slightly better than in conventional microscopes. If the aperture diameter is in the first minimum of the diffraction disk (i.e. in the first black ring), the resolution in the focal plane is no longer better than in the non-confocal case, whereas the signal intensity is then almost at its maximum. This value is often preset in the software of commercial confocal microscopes. It is called an Airy Unit (AU), after the English terms Airy disk (= diffraction disk ) and unit (= unit of measurement).
As is generally the case with light microscopes, the resolution in the focal plane is better than along the optical axis ( anisotropic resolution). The table shows the formulas for calculating the half-width of the point spread function.
Ø> 1 AU | Ø <0.25 AU | Objective NA = 1.4 / n = 1.518 for Ø = 1 - 0.25 AU (λ = 500 nm) |
confocal volume isosurface of equal intensity |
|
---|---|---|---|---|
FWHM lateral | 182-132 nm |
![]() |
||
FWHM axial | 473 - 344 nm | |||
Formulas for calculating the half width (FWHM) of the point spread function (PSF) with a diameter Ø of the detection pinhole of> 1 AU (column 2) and <0.25 AU (column 3). NA: numerical aperture of the objective; λ: wavelength of light; n: refractive index of the immersion medium (1 for air). Column 3 contains an example of a high-quality oil immersion objective, column 4 a representation of the volume from which the signal arrives at the detector (optical axis vertical).
|
The relationships described only apply to ideal optical conditions. One of these conditions is a completely uniform illumination of the rear focal plane of the objective by the excitation light, since only then is an ideal diffraction disk created in the specimen. However, the brightness cross-section of a laser beam shows approximately a Gaussian distribution . In order to minimize the negative effect, the rear pupil of the objective is overexposed, i.e. the laser beam is widened so much that the outer areas are cut off and the remaining cross-section has fewer differences in brightness. If it is widened so much that 50% of the light can still pass through, the loss of resolution is still around 8%; if 30% still penetrate, the loss is 5%. Furthermore, when examining fluorescence, there is a difference between the excitation and detection wavelength ( Stokes shift ), which leads to a further loss of resolution. The mean wavelength is approximated to .
A further loss of resolution can be caused in the specimen if the refractive index of the embedding medium or the cover glass thickness deviates from the values intended for the objective and this leads to spherical aberrations .
Axial limitation of the signal and positioning accuracy
The slight improvement in resolution hardly justifies the increased effort and the associated costs. Rather, the decisive advantage of confocal microscopes is the ability to record optical sections, because the intensity of the signal decreases with the fourth power of the distance to the focal plane (1 / distance 4 ). The effect can be explained using the example of a reflective surface with reflection. In conventional reflected light microscopes, the exact position of the surface cannot be determined because the amount of light reflected is the same in the levels above and below. In the confocal microscope, however, light is only detected when the reflective surface is in the area of the focal plane. As a result, the accuracy of a position determination is very high. The contrast in fluorescent specimens is also improved since no fluorescence from other levels reaches the detector.
The accuracy with which a position can be determined is considerably better than the achievable resolution, since the center of a brightness maximum can be determined very precisely (see figure). As a result, the achievable resolution is not undershot, since it cannot be determined whether the recorded signal originates from one or more closely spaced structures. This restriction is irrelevant if the height of surfaces is to be measured in materials research . Such a height measurement is therefore not limited by the resolution. The limiting factor is the uncertainty with which the position of the maximum intensity along the optical axis can be determined. The uncertainty is primarily limited by the system noise and is only a few nanometers in a well-designed confocal microscope when using a high-aperture objective.
Related procedures
Non-microscopic confocal techniques
Confocal techniques are also used outside of microscopy, for example for chromatic confocal distance measurements . The article Confocal Technology gives an overview .
In medicine, confocal endomicroscopy is used as a method of endoscopy .
Other laser scanning microscopes
- see also: Laser Scanning Microscopes
To a certain extent, the precursors of confocal laser scanning microscopes are flying spot microscopes . As with the confocal point scanners, an illumination point is guided over the specimen. However, this is not necessarily diffraction-limited. A detection pinhole is also missing. In early devices, the point of illumination was often generated by a Braun tube , and after the development of the laser also by a focused laser beam. Such devices were the first laser scanning microscopes.
Other laser scanning microscopes, however, were only built after the development of the confocal laser scanning microscope. 4Pi microscopes and STED microscopes are special confocal microscopy that enables improved resolution. Multiphoton microscopy , on the other hand, is a non-confocal laser scanning method, since no pinhole diaphragms with confocal focal points are required. A signal is only generated here when two or more photons arrive at the focal point at the same time, hence the name. The three methods mentioned are often built in as an accessory to a confocal laser scanning microscope.
history
1940: a slit lamp system for documenting eye examinations
The ophthalmologist Hans Goldmann , director of the University Eye Clinic in Bern , Switzerland , struggled with the problem that slit lamps only ever sharply focus on a very limited part of the eye ( cornea or part of the lens ). While an observer could compose a continuous image in his mind, a photographic representation was always limited to a narrow area. Goldmann developed a device for "slit lamp photography and photometry", which was manufactured by the local company Haag-Streit .
The sharp portion of the image was projected onto film through a lens . A gap-shaped aperture in front of the film prevented blurred parts of the image from being photographed. The mechanism for moving the illumination slit was firmly connected to the film drum via a disk: if the slit of the sharp image moved over the eye, the film in the drum would rotate accordingly behind the slit diaphragm.
The lighting through a gap corresponds roughly to a light disk , so the lighting is not focused on a line, as in later confocal line scanners. The observation axis in Goldmann's apparatus, however, was approximately at a 45 ° angle to the illumination axis, so that - viewed from the detector - only one line (and not the levels below or above) was illuminated. The function of the gap in front of the camera corresponded to the slit diaphragm in front of the detector of a confocal line scanner, so that the system was subsequently referred to as confocal.
Goldmann's work was cited in the 1970s by DM Maurice who developed a line scanning confocal microscope for ophthalmology. Goldmann's apparatus also mention historical retrospectives.
1943, 1951: confocal microscopes for spectrophotometry
Published in 1943 Zyun Koana a work on a confocal micro photometry system. A figure shows the scheme of a confocal transmission beam path: The illumination passes through two lenses and the excitation pinhole to another lens (corresponding to the condenser in normal light microscopes), from which it is focused into the specimen. The light passes through the specimen and is focused on a detection pinhole by another lens (corresponding to the objective). There is no translation or summary in a western language of this Japanese work. Koana (1907–1985) later became known for developing camera lenses in collaboration with the Nikon company .
Hiroto Naora, a Koana collaborator, published a paper in Science magazine in 1951 . He focused a pinhole in the illumination beam path, reduced 2000 times, into the specimen. A second pinhole in the intermediate image plane minimized stray light during the exposure. The aim was to illuminate small areas in cell nuclei in which the DNA was detected with the Feulgen stain in order to quantitatively determine the amount of DNA. The microscope did not produce any images; instead, the intensity and the wavelengths of the light transmitted by the specimen were measured ( spectrophotometry ). The two perforated diaphragms avoided scattered light and thus improved the measurement accuracy. The illumination spot produced had a diameter of 1-5 μm and was therefore not diffraction-limited. The specimen was also not scanned.
1955, 1957: the first point scanner

The first point-scanning confocal microscope was developed by Marvin Minsky in 1955 and a patent applied for in 1957. He wanted to examine sections of the brain and was struggling with severe scatter in this dense tissue. Since the bright scattered light prevented the actual structures from being recognized, he looked for a way to reduce this and found it in the confocal principle.
The microscope had a horizontal, fixed beam path (see diagram) and a zirconium - arc lamp as a light source. The opening of the first pinhole was focused into the specimen through a 45x dry objective. The specimen was placed between two cover glasses and clamped into a movable device that was moved in the x and y directions for scanning (“stage scanning”). The second lens of the same type picked up the light that had passed through and passed it through the second pinhole to the detector. Minsky never used oil immersion because he feared that the viscosity could lead to problems with the movement of the specimen and that the lower penetration depth of the oil immersion objectives would not be sufficient. The system was able to resolve points less than a micrometer apart.
The detector was a photomultiplier and the display device was a radar screen ( cathode ray tube ) with an image display time of about ten seconds. This was also the time it took to rasterize an image. Digitization or photographic documentation was not built in, and no images have survived. Minsky later cited the lack of documentation capabilities as one reason why it took another 30 years for the idea of the confocal microscope to take hold.
Minsky did not write any scientific papers mentioning the confocal microscope. His brother-in-law, a patent attorney , found the device interesting, and so it was the only contemporary documentation in a patent application . This also describes the structure of a second version in which light passes through the specimen, then hits a mirror and is reflected back by this (see figure). He also mentioned the possibility of scanning along the optical axis (z-direction), which he did not realize either.
1966: "Device for the optical scanning of microscopic objects"
Less well known is the development of several approaches for confocal scanning microscopes by Klaus Weber, employee of Ernst Leitz GmbH in Wetzlar . As with Koana and Naora, the goal was not to create an image, but to measure the signal intensity in small sections of the specimen.
Weber envisaged three alternative options for scanning "for synchronous scanning on the lighting and observation side":
- A classic Nipkow disk (each with only one spiral made of pinhole diaphragms) came into the plane of the luminous field diaphragm and a second, synchronously running disk into the intermediate image plane (see left figure).
- In each of the luminous field and the intermediate image plane, there was a movable pinhole, which was electrically moved synchronously in one direction (x-axis). Scanning along the y-axis was done by moving the object or also by moving the pinhole.
- This "particularly advantageous arrangement" used a tilting mirror that moved the image of the illumination pinhole ("light spot stop") as a "light spot" over the specimen, and over which the observation beam path to the detection pinhole ("field stop") also ran. The specimen was stationary and was scanned by shifting the beam path (beam scanning).
Walter again suggested several versions of the tilting mirror variant. Either the illumination and observation light should run over the same side of the mirror, or, "particularly useful", the observation beam path ran over the rear side, which was also mirrored, so that the illumination and observation beam paths were separate, but still synchronized, so that the one in the specimen The light spot generated was always mapped onto the detection pinhole. For this solution, the patent application shows a version for transmitted light and one for incident light reflection microscopy (see figures). The possibility of using the electrical signal from the detector to create an image, as with the Minsky microscope, was not mentioned in the patents.
It is unclear whether the devices proposed by Weber were actually built and whether his ideas had an influence on further developments. The first patent citing Weber's US patent was filed in 1980.
Weber was not the first to have the idea of using a Nipkow disk in microscopes. As early as 1951, a system was presented in which a classic Nipkow disk was used to break down a fluorescence image in such a way that the brightness of the individual pixels could be measured one after the other by a photomultiplier. So it was not a confocal imaging system, but a "microfluorometric scanner".
1967: the first imaging confocal microscope with a Nipkow disk
In the 1960s, the Czechoslovak Mojmír Petráň from the Medical Faculty of the Charles University in Pilsen developed the tandem scanning microscope described above . It was the first confocal microscope that was offered for sale: on the one hand by a small company in Czechoslovakia and on the other hand in the USA by Tracor-Northern (later Noran).
The trained doctor Petráň visited the research group of Robert Galambos at Yale University in New Haven (Connecticut, USA) in 1964 . They thought about how unfixed, unstained nerve cells in the brain could be observed and developed the concept during this stay. The following year Petráň and Milan Hadravský built the first prototype in Pilsen.
The Czechoslovak patent was filed in 1966 by Petráň and Hadravský. In 1967 a first scientific publication appeared in the journal Science , which contained data and images obtained with the microscope. The authors were M. David Egger from Yale University and Petráň. The footnotes to this paper say that Petráň designed the microscope and directed its construction and that he was at times a research associate at Yale University. In 1968 another work appeared in which Hadravský and Galambos were authors. The theory and technical details of the microscope were described here. In 1970 the US patent applied for in 1967 was granted. It also contains a version of the microscope for transmitted light: the specimen is illuminated through a lens and observed through another, identically constructed. However, it is unclear whether this variant was actually built.
At the annual meeting of the European Light Microscopy Initiative (ELMI) in 2011 Petráň and Hadravský were honored for their services, and in 2012 both were named by the European Capital of Culture project “Plzeň 2015” and “Pilsen icons” in their hometown. Hadravský was given this honor in memoriam .
It was not until 1986 that the tandem scanning microscope was used to examine the eye . After successful ex vivo tests, scientists at Georgetown University in the USA further developed the device so that it could also be used on patients. A commercial version was developed by Tandem Scanning Corporation, Inc. The device contained an additional lens, the movement of which changed the plane of focus in the eye without having to move the lens.
1969: the first confocal laser scanning microscope
Egger was involved in a further development. Together with Paul Davidovits , also at Yale University, he published two papers in 1969 and 1971 on the first confocal microscope that worked with laser light, a point scanner. This device was also intended for reflected light reflection microscopy, in particular for the observation of nerve tissue. As early as 1969 the authors speculated about the use of fluorescent dyes for " in vivo " investigations. They cited the Minsky's patent and thanked Steve Baer for suggesting using a laser with 'Minsky's microscope', as well as Galambos, Hadravsky, and Petráň for discussions that led to the development of the microscope. Baer was a PhD student at the Albert Einstein School of Medicine in New York City , where he developed a line scanning confocal microscope. As a motivation for the new development, Egger and Davidovits stated in the second work that in the tandem scanning microscope only 10 −7 of the illumination in the eyepiece contributed to the formation of the image and that the image quality was therefore insufficient for most biological investigations.
A helium-neon laser with a wavelength of 633 nm and a power of 5 mW was directed onto a semitransparent mirror and reflected from this to the objective. A simple lens with a focal length of 8.5 mm served as the objective . In contrast to all earlier and most later developments, the specimen was scanned by moving this lens (objective scanning), which shifted the focal point accordingly. Reflected light went back to the semitransparent mirror, and the transmitted portion was guided by another lens to the detection pinhole, behind which a photomultiplier was located. The signal was displayed by the cathode ray tube of an oscilloscope with the cathode ray moving in synchronism with the lens. Special equipment was able to take Polaroid photos of the ad, three of which are reproduced in the 1971 publication. Laser light is basically linearly polarized . In the first of the two publications, an analyzer (a polarization filter ) in front of the detection pinhole prevented light that was reflected inside the microscope from reaching the detector. A λ / 4 plate between the objective and the specimen ensured that the light reflected by the specimen was shifted by a total of 90 ° in relation to the laser polarization so that it passed through the analyzer. In the microscope version of the second publication, however, these two components were missing.
Davidovits, Egger and Marvin Minsky received the RW Wood Prize of the Optical Society of America in 2000 for contributions to the development of the confocal microscope.
1977–1985: Point scanner with laser and rastering by specimen movement (stage scanning)
Colin JR Sheppard and A. Choudhury of Oxford published a theoretical analysis of confocal microscopy and laser scanning microscopy in 1977. This work is probably the first publication to contain the term “confocal microscope”.
In 1978, the brothers Christoph Cremer and Thomas Cremer in Heidelberg designed a confocal laser scanning microscope for fluorescence excitation with electronic autofocus . They wrote: "Due to its special display options, the laser scanning microscope process could become a valuable addition to conventional light microscopic and scanning electron microscopic processes." They also suggested laser point illumination using a "4π point hologram ".
In 1978 and 1980, the Oxford group around Sheppard and Tony Wilson described a reflected-light confocal microscope with stage-scanning, laser illumination and photo-multipliers as detectors. The specimen could not only be moved in the focal plane, but also along the optical axis, which made it possible to record optical serial sections. The advantages of the device could be shown particularly convincingly on integrated electronic circuits . The term and the method of "optical sectioning" is, however, older. As early as 1930, Francis Lucas showed optical serial cuts that he produced on film with a UV light microscope.
Fred Brakenhoff and co-workers proved in 1979 that the theoretical advantages of the optical sections and the improvement in resolution can actually be achieved. In 1985, the group published the first convincing images on cell biology issues, which they took with an advanced confocal microscope. Other biological applications followed shortly thereafter by other groups.
In the meantime, IJ Cox and Sheppard from the Oxford group published the first work on a confocal microscope connected to a computer in 1983.
The first commercial confocal laser scanning microscope, the SOM-25 stage scanner, was offered from 1982 by Oxford Optoelectronics (taken over by BioRad via intermediate steps); it was based on the design of the Oxford Group.
From 1985: laser point scanner with beam scanning
Most of the confocal laser scanning microscopes developed to date belonged to the stage scanning type: the point of illumination was immobile and the stage with the specimen was moved in the x and y directions in order to scan the objects in the focal plane. This procedure was slow and sensitive to shock. Minsky had already mentioned the possibility of swiveling a scanning light beam over the immobile specimen instead, but discarded this due to technical difficulties.
In the mid-1980s, WB Amos, JG White and colleagues in Cambridge developed the first confocal beam scanning microscope ( beam , English for ray, light beam) in which the specimen stood still and the point of illumination was moved instead. This made it possible to record four images per second with 512 lines each. The idea of beam scanning was adopted from flying spot microscopes . A second important new development was a greatly enlarged intermediate image plane through a beam path that was one to two meters longer. As a result, the intermediate image generated was 80 times larger than through the lens enlargement, with the result that an ordinary iris diaphragm with a diameter of about one millimeter could be used for the pinhole , in contrast to the pinhole diaphragms in earlier systems, which were only a few dozen micrometers in size. This made the adjustment much easier.
First photographs were taken with long-term lighting with film before a digital camera was installed. Images from various biological specimens were significantly better compared to normal fluorescence microscopy. Such images were first published in 1987. Another device improvement made it possible for the first time to zoom into the specimen, that is, to select a sub-area for an enlarged display or a faster image. Zeiss , Leitz and Cambridge Instruments had no interest in commercial production. However, the Medical Research Council (MRC) agreed to fund the development of a more compact prototype. Eventually Bio-Rad did the design and software for computer control was developed. The device came onto the market as the MRC 500, the successor was called the MRC 600. This device was also the basis for the first two-photon fluorescence microscope developed at Cornell University in the United States and published in 1990 .
At the same time there was a further development at Stockholm University , which at about the same time led to a commercial device that was sold by the Swedish company Sarastro. This was taken over by Molecular Dynamics, a US company, in 1990, but production eventually ceased. In Germany, Heidelberg Instruments , founded in 1984, developed a confocal laser scanning microscope that was developed less for biomedical than for industrial applications. This device was taken over and further developed by Leica Lasertechnik in 1990 . Zeiss already had a non-confocal flying-spot laser scanning microscope on the market, which was expanded into a confocal microscope. A 1990 report that mentions "some" manufacturers listed the following: Sarastro, Technical Instrument, Meridian Instruments, Bio-Rad, Leica, Tracor-Northern and Zeiss.
New developments with Nipkow disc
The many mirrors and prisms of the tandem scanner, which precisely coordinate and adjust the excitation pinhole diaphragms and detection pinhole diaphragms in the beam path, is difficult; The fluttering of the thin Nipkow disk also leads to adjustment difficulties. Egger and Petráň already proposed that the excitation and detection beam path be guided through the same pinhole diaphragms (“one-sided Nipkow disk”). However, they rejected this approach, since the reflection of the excitation light on the surface of the pane could not be separated from the detection beam path. Albert Frosch and Hans Erdmann Korth were awarded a US patent for IBM in 1975 , in which this problem was addressed by tilting the Nipkow disk.
With further improvements, this idea became the basis for a patent filed in 1988 by Gordon S. Kino and Guoqing Xiao, Stanford University , for a single-sided Nipkow disk microscope for reflected light reflection microscopy. The device was intended for the measurement of semiconductors . The advantages were a lower susceptibility to vibrations and a simplified adjustment. In addition, the Nipkow disk could now be moved transversely to the beam path, so that apertured diaphragms of different sizes could be attached in different tracks between the disk center and the edge. This enabled the size of the aperture diaphragm to be adapted to the resolution of different objectives or to the different degrees of reflection from different regions of the specimen.
To reduce the reflection directly from the Nipkow disk to the detector, a blackened disk inclined by 5 ° to the optical axis was installed. After the light source came a polarizer and behind it a semitransparent mirror that reflected to the Nipkow disk. Between the disk and the lens there was a quarter wave plate , on the return path of the light, directly in front of the detector, there was an analyzer . A larger part of the light was lost, but the light reflected directly from the Nipkow disk was effectively blocked by the analyzer.
Ichihara and colleagues published the first paper on the Yokogawa spinning disk system in 1996 (see above ).
Web links
- Optical Microscopy Primer (English) Extensive collection of links to detailed descriptions of microscopy, including virtual confocal microscopes
- Confocal Laser Scanning Microscopy Introduction to Confocal Microscopy from Zeiss (PDF file, 884 KB)
literature
further reading
- Michael Volger: Light microscopy - theory and application. Irene K. Lichtscheidl, University of Vienna (Ed.), Online edition February 29, 2008, PDF file at: univie.ac.at . (Treatise on light microscopy, pp. 174–220 on fluorescence and confocal microscopy.)
- Michiel Müller: Introduction to Confocal Fluorescence Microscopy (Tutorial Texts in Optical Engineering) . 2nd Edition. SPIE Press, 2006, ISBN 0-8194-6043-5 . Publisher website (Introduction to Confocal Fluorescence Microscopy.)
- Guy Cox: Optical Imaging Techniques in Cell Biology . 1st edition. CRC Press, Taylor & Francis Group, Boca Raton FL 2006, ISBN 0-8493-3919-7 . (2nd edition. 2012, ISBN 978-1-4398-4825-8 ) (General introduction to light microscopy, with chapters specifically on confocal microscopy and digital images, aberrations, fluorescence, etc.)
- James Pawley (Ed.): Handbook of Biological Confocal Microscopy . 3. Edition. Springer Science and Business Media, 2006, ISBN 0-387-25921-X . ( The reference work for confocal microscopy in the life sciences, less intended for beginners.)
Individual evidence
- ↑ a b c d Guy Cox: Optical Imaging Techniques in Cell Biology . 1st edition. CRC Press, Taylor & Francis Group, Boca Raton FL 2006, ISBN 0-8493-3919-7 , pp. 57-75 .
- ^ A b Colin JR Sheppard, David M. Shotton: Confocal Laser Scanning Microscopy . In: Royal Microscopical Society Microscopy Handbooks . tape 38 . BIOS Scientific Publishers Limited, Oxford, UK 1997, ISBN 1-872748-72-4 , pp. 37, 39-42 .
- ↑ Hybrid detector R10467U-40 ( page no longer available , search in web archives ) Info: The link was automatically marked as defective. Please check the link according to the instructions and then remove this notice. from the Hamamatsu Photonics KK website, accessed December 4, 2012.
- ↑ Leica HYD for Confocal Imaging. (PDF; 1.9MB) Leica Microsystems, accessed on February 9, 2016 .
- ↑ The HPM-100-40 Hybrid Detector. (PDF; 1.4MB) Becker & Hickl GmbH, accessed on February 9, 2016 .
- ↑ PMA Hybrid Series | PicoQuant. PicoQuant GmbH, accessed on July 14, 2017 .
- ↑ Confocal microscopes with stage scanning are offered by several manufacturers (e.g. MicroTime from PicoQuant , DeltaMyc from Horiba ).
- ↑ a b c P. Davidovits, MD Egger: Scanning laser microscope for biological investigations. In: Applied optics. Volume 10, Number 7, July 1971, pp. 1615-1619, ISSN 0003-6935 . PMID 20111173 . doi: 10.1364 / AO.10.001615
- ↑ a b MicroTime from PicoQuant , website of the manufacturer, accessed on July 14, 2017.
- ↑ DeltaMyc from Horiba , website of the manufacturer, accessed on July 14, 2017.
- ^ Alba FCS , manufacturer's website, accessed January 26, 2012.
- ↑ Confocal Raman microscope from Witec ( Memento of the original from December 22, 2012 in the Internet Archive ) Info: The archive link was inserted automatically and has not yet been checked. Please check the original and archive link according to the instructions and then remove this notice. Manufacturer's website, accessed January 26, 2012.
- ↑ a b c d e f g h i j k l m n o p Guy Cox: Optical Imaging Techniques in Cell Biology . 1st edition. CRC Press, Taylor & Francis Group, Boca Raton FL 2006, ISBN 0-8493-3919-7 , pp. 115-122 .
- ↑ Zeiss website for the Axio CSM 700 true color confocal microscope , also available there as a PDF file, accessed on January 26, 2012.
- ↑ a b c d J. C. Erie: Corneal wound healing after photorefractive keratectomy: a 3-year confocal microscopy study. In: Transactions of the American Ophthalmological Society. Volume 101, 2003, pp. 293-333, ISSN 0065-9533 . PMID 14971584 . PMC 1358995 (free full text).
- ↑ a b c Egger MD, Petrăn M: New reflected-light microscope for viewing unstained brain and ganglion cells . In: Science . tape 157 , no. 786 , July 1967, p. 305-7 , doi : 10.1126 / science.157.3786.305 , PMID 6030094 .
- ^ A b Mojmír Petráň, Milan Hadravský: Method and arrangement for improving the resolving power and contrast. online at Google Patents, filed November 4, 1967, issued June 30, 1970.
- ↑ a b c Mojmír Petráň, Milan Hadravský, M. David Egger, Robert Galambos: Tandem-Scanning Reflected-Light Microscope . In: Journal of the Optical Society of America . tape 58 , no. 5 , 1968, p. 661-664 , doi : 10.1364 / JOSA.58.000661 .
- ↑ Heike Schmidt, Jürgen Valentin: Confocal measurement - 3D surface measurement using a microscope. In: Practice Profiline. November 2006. PDF file
- ↑ Andromeda iMIC - High-speed live cell imaging with Confocal resolution. Brochure from Till Photonics. Available online as a PDF file ( Memento of the original dated August 16, 2012 in the Internet Archive ) Info: The archive link was inserted automatically and has not yet been checked. Please check the original and archive link according to the instructions and then remove this notice.
- ↑ a b c B. Amos, G. McConnell, T. Wilson: Confocal microscopy. In: E. Egelman (Ed.): Comprehensive Biophysics. Elsevier, Amsterdam 2012.
- ^ G. Cox, CJ Sheppard: Practical limits of resolution in confocal and non-linear microscopy. In: Microscopy research and technique. Volume 63, Number 1, January 2004, pp. 18-22, ISSN 1059-910X . doi: 10.1002 / Jemt.10423 . PMID 14677129 .
- ↑ a b c Stefan Wilhelm, Bernhard Gröbler, Martin Gluch, Hartmut Heinz: The confocal laser scanning microscopy . 09/03 edition. Carl Zeiss microscope systems, Jena 2003 ( PDF; 884kB ).
- ↑ a b c d e f W. B. Amos, JG White: How the confocal laser scanning microscope entered biological research. In: Biology of the cell / under the auspices of the European Cell Biology Organization. Volume 95, Number 6, September 2003, pp. 335-342, ISSN 0248-4900 . PMID 14519550 . (Review).
- ↑ VDI / VDE 2655-1.2: Optical measurement technology on microtopographies; Calibration of confocal microscopes and depth setting standards for roughness measurement. Content draft (PDF; 112 kB)
- ↑ M. Françon: Introduction to the newer methods of light microscopy . G. Braun Verlag, Karlsruhe 1967, p. 253-256 .
- ↑ a b Hans Goldmann: Slit lamp photography and photometry . In: Ophthalmologica . tape 98 , no. 5/6 , 1939, pp. 257-270 , doi : 10.1159 / 000299716 . Note: Volume 98 is assigned to the year 1939, but January 1940 is given as the date of publication on the first page of the article.
- ↑ a b c d e f g h Colin JR Sheppard: Confocal Microscopy. The Development of a Modern Microscopy . In: Imaging & Microscopy . November 3, 2009 ( online [accessed November 17, 2012]).
- ↑ a b Barry R. Masters: Confocal Microscopy And Multiphoton Excitation Microscopy. The Genesis of Live Cell Imaging . SPIE Press, Bellingham, Washington, USA 2006, ISBN 978-0-8194-6118-6 , pp. 120-121 .
- ↑ Barry R. Masters: Confocal Microscopy And Multiphoton Excitation Microscopy. The Genesis of Live Cell Imaging . SPIE Press, Bellingham, Washington, USA 2006, ISBN 978-0-8194-6118-6 , pp. 126 .
- ↑ Zyun Koana: 微小 部 濃度 計 に 關 す る 諸 問題 . In: Journal of the Illumination Engineering Institute . tape 26 , no. 8 , 1942, pp. 371-385 . The work ( online PDF file, 19020 kilobytes) Figure 1b of the work (p. 373) shows the scheme of a confocal transmission beam path
- ↑ Dr. Zyun Koana Special Exhibition at the University of Tokyo ( Memento of the original from April 2, 2015 in the Internet Archive ) Info: The archive link was inserted automatically and has not yet been checked. Please check the original and archive link according to the instructions and then remove this notice. , Michio Akiyama's website, accessed January 8, 2013.
- ↑ Hiroto Naora: Microspectrophotometry and cytochemical analysis of nucleic acids. In: Science. Volume 114, Number 2959, September 1951, pp. 279-280, ISSN 0036-8075 . PMID 14866220 . doi: 10.1126 / science.114.2959.279
- ^ Marvin Minsky: Microscopy Apparatus. U.S. Patent 3,013,467 , filed November 7, 1957, issued December 19, 1961.
- ↑ a b c d Marvin Minsky : Memoir on inventing the confocal scanning microscope . In: Scanning . tape 10 , no. 4 , 1988, pp. 128-138 , doi : 10.1002 / sca.4950100403 .
- ↑ a b c Inventor: Dr. Klaus Weber, Wetzlar, applicant Ernst Leitz GmbH: Device for optical scanning of microscopic objects German Patent Office, Offenlegungsschrift 1472293 (online) , filing date August 10, 1966, publication date January 23, 1969.
- ^ A b Klaus Weber, Wetzlar, assignor to Ernst Leitz GmbH: Device for optically scanning the object in a microscope. U.S. Patent 3518014 ( online at Google Patents ), filed August 7, 1967, issued June 30, 1970.
- ↑ Barry R. Masters: Confocal Microscopy And Multiphoton Excitation Microscopy. The Genesis of Live Cell Imaging . SPIE Press, Bellingham, Washington, USA 2006, ISBN 978-0-8194-6118-6 , pp. 78-79 .
- ↑ RC MELLORS, R. SILVER: A micro-fluorometric scanner for the differential detection of cells; application of exfoliative cytology. In: Science. Volume 114, Number 2962, October 1951, pp. 356-360, ISSN 0036-8075 . PMID 14883859 .
- ↑ a b c d e f g Barry R. Masters: Confocal Microscopy And Multiphoton Excitation Microscopy. The Genesis of Live Cell Imaging . SPIE Press, Bellingham, Washington, USA 2006, ISBN 978-0-8194-6118-6 , pp. 88-96 .
- ↑ 11th annual meeting of the European Light Microscopy Initiative (ELMI), 2011, Meeting's Program ( Memento from August 31, 2013 in the Internet Archive )
- ↑ Plzeň 2015 selects Pilsner Ikonen 2012. In: bbkult.net. Centrum Bavaria Bohemia , accessed on February 9, 2016 .
- ^ A b P. Davidovits, MD Egger: Scanning laser microscope. In: Nature. Volume 223, Number 5208, August 1969, p. 831, ISSN 0028-0836 . PMID 5799022 . doi: 10.1038 / 223831a0
- ↑ Barry R. Masters: Confocal Microscopy And Multiphoton Excitation Microscopy. The Genesis of Live Cell Imaging . SPIE Press, Bellingham, Washington, USA 2006, ISBN 978-0-8194-6118-6 , pp. 124-125 .
- ↑ a b c d e f Shinya Inoué: Foundations of Confocal Scanned Imaging in Light Microscopy . In: James Pawley (Ed.): Handbook of Biological Confocal Microscopy . 3. Edition. Springer Science and Business Media LLC, 2006, ISBN 978-0-387-25921-5 , Chapter 1, pp. 1-19 .
- ^ CJR Sheppard, A. Choudhury: Image Formation in the Scanning Microscope. In: Optica Acta: International Journal of Optics. 24, 1977, pp. 1051-1073, doi: 10.1080 / 713819421 .
- ↑ C. Cremer, T. Cremer: Considerations on a laser-scanning-microscope with high resolution and depth of field. In: Microscopica acta. Volume 81, Number 1, September 1978, pp. 31-44, ISSN 0044-376X . PMID 713859 .
- ↑ FF Lucas: THE ARCHITECTURE OF LIVING CELLS-RECENT ADVANCES IN METHODS OF BIOLOGICAL RESEARCH-OPTICAL SECTIONING WITH THE ULTRA-VIOLET MICROSCOPE. In: Proceedings of the National Academy of Sciences . Volume 16, Number 9, September 1930, pp. 599-607, ISSN 0027-8424 . PMID 16587612 . PMC 526698 (free full text).
- ^ IJ Cox, CJ Sheppard: Scanning optical microscope incorporating a digital frame store and microcomputer. In: Applied optics. Volume 22, Number 10, May 1983, p. 1474, ISSN 0003-6935 . PMID 18195988 .
- ↑ Brent Johnson: Image Is Everything . In: The Scientist . February 1, 1999 ( online [accessed November 30, 2012]).
- ^ Website of Heidelberg Instruments GmbH. Retrieved June 4, 2014 .
- ↑ Diana Morgan: Confocal Microscopes Widen Cell Biology Career Horizons . In: The Scientist . July 23, 1990 ( online [accessed November 30, 2012]).
- ^ Albert Frosch, Hans Erdmann Korth: Method of increasing the depth of focus. U.S. Patent 3,926,500. at Google Patents
- ↑ Gordon S. Kino, Guoqing Xiao: Scanning confocal optical microscope including an angled apertured rotating disk placed between a pinhole and an objective lens. U.S. Patent 4,927,254, filed July 29, 1988, issued May 22, 1990 on- line from Google Patents
- ↑ Shinya Inoué: Foundations of Confocal Scanned Imaging in Light Microscopy . In: James Pawley (Ed.): Handbook of Biological Confocal Microscopy . 3. Edition. Springer Science and Business Media LLC, 2006, ISBN 0-387-25921-X , Chapter 1, pp. 1-19 .