Förster resonance energy transfer
The Förster resonance energy transfer ( FRET for short ), sometimes also (incorrectly) called fluorescence resonance energy transfer , is a physical process of energy transfer named after Theodor Förster . As part of the Förster resonance energy transfer, the energy of an excited dye , also called donor, is transferred to a second dye, also called acceptor. The energy is radiation-free and therefore not exchanged via a release ( emission ) and absorption ( absorption ) of light particles ( photons ). For example, the light-gathering complex of organisms engaged in photosynthesis is based on the Förster resonance energy transfer . In biochemistry and cell biology , the Förster resonance energy transfer is used in particular with the use of fluorescent dyes as an “optical nanometer scale”, since the intensity depends, among other things, on the distance between donor and acceptor and can be observed in the range of up to 10 nm.
history
The history of the discovery and research of Förster resonance energy transfer can be traced back a long way from Theodor Förster's essay “Intermolecular Energy Migration and Fluorescence”, which was published in 1948 in the Annalen der Physik . As early as the early 19th century, Hans Christian Ørsted , Michael Faraday , James Clerk Maxwell and Heinrich Hertz provided important basic knowledge for the later scientific description of this phenomenon .
The first experimental evidence of energy transfer between two molecules came from G. Cario and James Franck in 1922 . In a mercury - thallium vapor mixture, they observed a characteristic emission of light from thallium, which they termed "sensitized fluorescence", after excitation with light of a wavelength that is specific for the excitation of mercury. Initial attempts to explain this energy transfer by Jean-Baptiste Perrin and Francis Perrin are based on a so-called near - field - dipole-dipole mechanism . Hartmut Kallman and Fritz London found a quantum mechanical explanation for this radiation-free energy transfer. These findings flowed into Francis Perrin's attempt to quantitatively describe this energy transfer. However, it was based on the misconception that the energy transfer decreases with the third and not the sixth power of the distance between the two molecules.
In 1946, shortly after the Second World War, Theodor Förster took part in the discussion on the quantitative description of radiation-free energy transfer. But it was not until his essay “Intermolecular Energy Migration and Fluorescence” from 1948 that it received a broad response in science.
physics

The Förster resonance energy transfer can be observed between two dyes that are present as molecules, complexes or nanoparticles . The energy of an excited donor dye is not given off in the form of a photon , but rather transferred to an acceptor dye without radiation via dipole-dipole interactions . While Coulomb's law applies to monopoles, the electrical dipole-dipole interaction is responsible for the energy transfer, which is why the transfer also depends on the sixth power of the distance. Descriptions of the Förster resonance energy transfer with the help of classical physics or quantum mechanics lead to comparable results. Accordingly, the excited donor D * induces an oscillation in the acceptor A. Another characteristic of the Förster resonance energy transfer is that the quantum mechanical property of the spin of the donor and acceptor dyes is retained. Therefore, the Förster resonance energy transfer usually occurs as a so-called singlet - singlet transfer:
In contrast, energy transfers, which only take place while maintaining the total spin of the donor and acceptor dye, occur as a Dexter energy transfer based on an exchange of electrons in the range of less than 2 nm.
The energy transferred to the acceptor dye can be given off again by the latter, for example in the form of radiation . In contrast, the transferred energy is no longer available to the donor dye for direct emission of radiation. The dwell time of the donor dye in the excited state also decreases. Macroscopically, a Förster resonance energy transfer expresses itself on the basis of the decrease in the radiation intensity and the fluorescence lifetime of the donor dyes and, in the case of acceptor dyes capable of radiation, an increase in the radiation intensity of the acceptor dyes .
Donor and acceptor are mostly fluorescent dyes, which is why the term fluorescence resonance energy transfer is often used as a synonym. However, fluorescence is not an essential requirement and is not involved in energy transfer. A Förster resonance energy transfer can also be observed between donors that are capable of other forms of radiation, such as phosphorescence , bioluminescence or chemiluminescence , and suitable acceptor dyes. Acceptor dyes may even be non-emissive and can lead to fluorescence quenching (dark quencher) .
FRET efficiency
The efficiency of the Förster resonance energy transfer , which can assume values between 0 and 1, is the ratio of the number of energy transfers and the number of donor excitations. It is also reflected in the Förster resonance energy transfer rate in relation to the sum of the rates for radiation emission of the donor dye , energy transfer and the forms of radiation-free degradation :
In order to enable the most efficient radiation-free energy transfer from the donor dye to the acceptor dye via the Förster resonance energy transfer, three essential criteria must be met:
- Distance: Donor dye and acceptor dye should only be a few nanometers apart.
- Spectrum: The radiation emission spectrum of the donor dye must overlap with the absorption spectrum of the acceptor dye.
- Orientation: Donor and acceptor dyes should have electronic oscillation planes that are as parallel as possible.
distance
The extent of the Förster energy transfer, represented as the transfer rate , depends on the radiation emission rate of the donor dye or its residence time in the excited state ( fluorescence lifetime ) and, in particular, on the distance between donor and acceptor dye . With a Förster resonance energy transfer, this is usually in the range of about 0.5 to 10 nm, the efficiency of the energy transfer decreasing with the sixth power of the distance between the two dyes.
The transfer rate is also determined by the Förster radius of the donor-acceptor dye pair. corresponds to the distance between the two dyes at which 50% of the energy is transferred:
In this way, the Förster resonance energy transfer differs from an energy transfer that is based on pure emission and absorption of radiation and that decreases with the square of the distance between donor and acceptor. It also differs from the Dexter electron transfer , which can be observed especially at very small distances (<0.5 nm) , which is based on an overlapping of the electron orbitals and decreases exponentially with the distance.
The criterion of the small distance between donor and acceptor means that the Förster resonance energy transfer is often used as an “optical nanometer measure”. Its use allows the distance between a donor and its acceptor to be measured in the near field, well below the resolution of conventional optical methods, such as microscopy . Therefore, the use of Förster resonance energy transfer has established itself as a valuable method, particularly in biochemistry and cell biology.
Spectra
A prerequisite for energy transfer is that the acceptor dye is capable of absorbing energy. For this purpose, the amount of energy to be transferred, which corresponds to the energy difference between the excited state and the ground state, must be in the range of the possible energy absorption of the acceptor dye. This is the case when the emission spectrum of the donor dye overlaps with the absorption spectrum of the acceptor dye. The size of the overlapping area of the spectra of donor and acceptor dye, represented as the integral , is proportional to the transfer rate and to the sixth power of the Förster radius :
- ,
where is the normalized radiation intensity of the donor dye at the wavelength and the extinction coefficient of the acceptor dye.
orientation
For an optimal energy transfer, donor and acceptor dyes should have electronic oscillation planes that are as parallel as possible. The transfer rate and the sixth power of the Förster radius of the donor-acceptor pair are proportional to the orientation factor :
- ,
where the angle between the emission dipole of the donor dye and the absorption dipole of the acceptor dye as well as and the angle between both dipoles and the connecting vector between donor and acceptor dye. can assume values between 0 and 4, whereby the value 0 is achieved with perpendicularity ( orthogonality ) of the dipoles and the value 1 with parallel and the value 4 with collinear arranged dipoles. For freely moving dyes, for example when investigating processes in solution, is . If, on the other hand, donor and acceptor dyes are fixed (e.g. on the same protein or in membranes), this value can deviate and the Förster resonance energy transfer can provide information about the change in the vibration levels and thus the position of both dyes relative to one another.
Measurement
Since the Förster resonance energy transfer is a radiation-free energy transfer, it cannot be directly detected and quantified. However, the consequences of an energy transfer, the decrease in radiation intensity and the fluorescence lifetime of the donor dye and, in the case of acceptor dyes capable of emitting radiation, the acceptor emission can be detected with the help of suitable instrumental methods. Fluorescence microscopes or fluorimeters , for example, are suitable for this purpose . Strictly speaking, in order to prove an energy transfer based on the Förster mechanism, there must also be an inverse proportionality between the energy transfer rate and the sixth power of the distance between the two dyes. In practice, however, especially in biochemistry, the type of energy transfer is rarely checked.
Measurement of the radiation emission of the donor
Since the Förster resonance energy transfer leads to a decrease in the radiation emission of the donor dye, its measurement is suitable for the detection of a resonance energy transfer. The FRET efficiency is quantified by measuring the radiation intensity of the donor dye in the absence and presence of the acceptor dye :
The radiation intensity can not only be determined in the form of a control experiment carried out in parallel in the absence of the acceptor. can also be determined in the presence of the acceptor, provided that it can be assumed that the distance between donor and acceptor dye is of an order of magnitude that is irrelevant for a Förster resonance energy transfer. This is used, for example, in biochemistry for proteins which are marked with dyes and which associate or dissociate in response to an external stimulus, for example a signal substance. Another method of determining whether the acceptor dye is present in spite of the presence of the acceptor dye consists in its destruction, for example with the aid of photo-bleaching . The photobleaching of the acceptor dye, which leads to an increase in the radiation intensity of the donor dye to a value without resonance energy transfer, is used in particular with immobilized samples, for example in fluorescence microscopy .
Measurement of the energy transfer to the acceptor
Another indication of a resonance energy transfer is the increase in the radiation intensity of the acceptor dye, provided it is capable of emitting radiation. In practice, however, the emission spectrum of the acceptor dye is often superimposed by the excitation spectrum and the emission spectrum of the donor dye. In addition, direct excitation of the acceptor dye by a radiation source used to excite the donor dye often cannot be avoided. However, these problems can be minimized by a specific selection of donor and acceptor dyes.
The problem of direct excitation of the acceptor dye by a radiation source used to excite the donor dye is eliminated when a chemi - or bioluminescent donor is used. In particular, bioluminescent dye donors, such as the luciferase of Renilla reniformis and its substrate coelenterazine , in combination with the green fluorescent protein (GFP) or its derivatives find the distance determination of proteins with the aid of bioluminescence resonance energy transfer application.
A further possibility of avoiding a falsification of results through direct excitation of the acceptor consists in a brief excitation of a donor dye with a long fluorescence lifetime and a time-delayed measurement of the acceptor fluorescence. Long-lived lanthanoids such as Tb 3+ , Dy 3+ , Eu 3+ or Sm 3+ , in particular, are used as donors for this process, which is also called time-resolved FRET . These also offer the advantage that their emission spectra, which consist of discrete peaks, hardly overlap that of the acceptor allophycocyanin .
The chemical or chromatographic detection of photolytic reaction products of the acceptor dye can also be used to calculate the FRET efficiency .
Measurement of the fluorescence lifetime
The dwell time of the donor dye in the excited state, which is reduced by the Förster resonance energy transfer, can be used by measuring its fluorescence lifetime for the detection of an energy transfer and for its quantification. To calculate the FRET efficiency , the fluorescence lifetime of the donor dye in the absence and presence of the acceptor dye is determined:
The fluorescence lifetime is usually determined as an imaging method with the help of fluorescence lifetime microscopy (FLIM). The measurement itself is done pixel by pixel. The fluorescence lifetime can be determined, for example, by measuring the decrease in fluorescence over time with the aid of the time- correlated single photon counting after an ultrashort, pulsatile excitation. Much more frequently, however, the measurement of the phase shift after an intensity-modulated excitation is used to determine the fluorescence lifetime ( phase fluorimetry ).
Occurrence and use
photosynthesis
Together with the Dexter energy transfer, the Förster resonance energy transfer is responsible for the function of the light-collecting complex in photosynthesis. The task of this complex, which consists of various membrane proteins , chlorophylls , carotenoids and phycobilins in the case of cyanobacteria and various algae , is the effective collection of light energy and its transmission to the reaction center of the light reaction . A gradual energy transfer from carotenoids or phycobilins via chlorophyll b to chlorophyll a in the core of the light-harvesting complex leads to an effective collection of energy from light of different wavelengths with the release and transfer of electrons . The Förster resonance energy transfer extends the absorption spectrum of the light-collecting complex to include wavelength ranges that chlorophyll a itself cannot use.
Bioluminescence
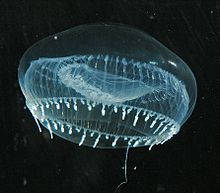
In nature, the Förster resonance energy transfer is used in the context of bioluminescence, the generation of light by living beings. An example of this is the Pacific jellyfish species Aequorea victoria . On the one hand, their light organs contain the photoprotein aequorin with its bound cofactor coelenterazine . This produces a blue light in isolated form and in the presence of Ca 2+ . On the other hand, the light organs contain the green fluorescent protein (GFP). Thanks to an efficient energy transfer from the aequorin to the green fluorescent protein, Aequorea victoria glows with a blue-green color.
Another example of the use of Förster resonance energy transfer in the animal kingdom is the bioluminescence of the sea feather species Renilla reniformis . With it, the energy transfer takes place from a luciferase , the Renilla luciferase (RLuc), to the green fluorescent protein.
Both based on the Förster resonance energy transfer fluorescent system of Aequorea victoria from aequorin and green fluorescent protein and that of Renilla reniformis from Renilla experimentally luciferase and green fluorescent protein are in biochemistry for the detection of protein-protein interactions used .
Semiconductor technology
When operating organic semiconductors as the active material in organic light-emitting diodes (OLEDs), the Förster resonance energy transfer plays a decisive role. Because the spectra of emission and absorption are not necessarily congruent in the wavelength range, a 4-level system arises "by itself", as is required for lasers. At the same time, a favorable coupling-out situation is achieved, since the emitted light is less strongly reabsorbed.
The energy transfer in organic light-emitting diodes, which are currently being developed as a possible alternative to the liquid crystal screen, is based on the Förster resonance energy transfer, the Dexter energy transfer and the direct generation of excitons between an excited host (donor) and a phosphorescent guest ( Acceptor). For example, carbazoles , polyphenylenes and fluorenes are used as host materials, while phosphorescent osmium , platinum and, in particular, iridium complexes are used as guest dyes .
biochemistry
In protein biochemistry and, based on this, in cell biology and pharmacology , the Förster resonance energy transfer is used analytically to prove protein-protein interactions and the interaction of proteins with other substances. In contrast to most other methods, such as co-immunoprecipitation , affinity chromatography , the yeast two-hybrid system and bimolecular fluorescence complementation , the measurement of the Förster resonance energy transfer allows the observation of protein-protein interactions in real time with a temporal resolution in the millisecond range.
Analysis of protein-protein interactions
In the context of the protein-protein interaction studies based on the measurement of the Förster resonance energy transfer, the protein pairs to be examined are marked with the help of pairs of fluorescent or other luminescent dyes, so that when the proteins to be examined interact, an increase in the Förster resonance energy transfer between the coupled dyes can be observed. These protein-protein interaction studies based on Förster resonance energy transfer are used, for example, to elucidate signal transmission paths in the cell and to search for new active substances with the help of high-throughput screening .
Various methods are available for labeling the proteins to be examined. The unselective direct chemical labeling, for example with the aid of fluorescein isothiocyanate (FITC) or tetramethylrhodamine isothiocyanate (TRITC), is particularly suitable for isolated proteins. A selective, albeit indirect, labeling consists in the use of fluorescence-labeled antibodies which are directed against the proteins to be examined. Marking with the help of simple molecular biological methods has established itself particularly in cell biology. In this way, derivatives of the green fluorescent protein (GFP), such as the donor-acceptor pair CFP-YFP, can also be coupled in vivo to the proteins to be examined. The bioluminescent Renilla luciferase is also used as a donor in combination with a GFP derivative as an acceptor in the context of bioluminescence resonance energy transfer studies. The disadvantage of these dyes is their size, which can influence the protein-protein interaction analysis. This problem can be circumvented with the help of low molecular weight compounds, such as fluorescein arsenical helix binder (FlAsH) and resorufin arsenical helix binder (ReAsH), which bind to specific amino acid sequences of the proteins to be investigated that have been inserted using molecular biological methods.
Analysis of protein conformational changes
If both the donor and the acceptor dye are coupled to one and the same protein molecule, the observation of an intramolecular Förster resonance energy transfer can also allow conclusions to be drawn about the conformation and conformational change of proteins. This principle is used by FRET-based biosensor proteins, such as the Ca 2+ indicator Cameleon and Epac for the detection of cyclic adenosine monophosphate (cAMP), which are subject to a conformational change in the presence of their substrate. The weakly fluorescent amino acids tyrosine and tryptophan , which occur endogenously in proteins, can also be used as acceptors in combination with suitable donors, such as terbium , for protein structure elucidation based on a Förster resonance energy transfer.
Molecular biology
In molecular biology, DNA -based methods using Förster resonance energy transfer are widely used. Dye-coupled DNA oligonucleotides are used here, in which an energy transfer can be observed by forming a duplex or, alternatively, an energy transfer is terminated when their tertiary structure is destroyed. These can be used as analytical tools in the polymerase chain reaction (PCR), hybridization , ligation , cleavage, recombination and synthesis of nucleic acids . They are also used in DNA sequence analysis , mutation analysis and determining the concentration of DNA and RNA.
Different dye-coupled oligonucleotides are used for this multitude of application areas. In addition to donors such as fluorescein , the red fluorescent rhodamine and related dyes as well as non-fluorescent dark quenchers are used as acceptor dyes . Although the latter do not allow an energy transfer to be identified on the basis of an increase in the acceptor emission, they offer the advantage of the possibility of a single-channel measurement of the donor fluorescence, which is not superimposed by an acceptor fluorescence.
Hybridization
The so-called hybridization probes (LightCycler probes) are among the molecular biological tools that use Förster resonance energy transfer and are used to identify DNA by means of hybridization . They consist of two non-complementary oligonucleotides, one dye of the donor-acceptor pair being coupled to the 3 'end of one and to the 5' end of the other oligonucleotide. If both oligonucleotides bind to a single DNA strand in close proximity, a Förster resonance energy transfer after donor excitation can be observed through a decrease in donor fluorescence and an increase in acceptor fluorescence. A modification of this method consists in the use of hybridization probes, which consist of mutually complementary DNA strands. They also allow the concentration of a target DNA to be determined, a decrease in energy transfer being observed due to competition between target DNA and complementary strand of the probe after hybridization.
Molecular beacons are also used in the context of hybridization analyzes . These consist of a loop-stem structure with a self-complementary stem at the 3 'and 5' ends, at which a donor fluorescent dye or a quencher are located, and a loop that is complementary to the target DNA. A decrease in energy transfer can be observed through an increase in donor fluorescence after excitation as a result of hybridization of the probe with a complementary target DNA and dissolution of the tertiary structure of the molecular beacons.
Polymerase chain reaction (PCR)
Particularly in the context of quantitative real-time PCR , DNA oligonucleotide-based probes are used to determine the concentration of the synthesized DNA by analyzing the Förster resonance energy transfer. In addition to hybridization probes and molecular beacons, hydrolysis probes (TaqMan probes) are used in particular . These consist of short DNA oligonucleotides which are complementary to the target DNA and to whose 5 'and 3' ends a donor fluorescent dye or a quencher is coupled. While the donor fluorescence is suppressed in the absence of sufficient amounts of complementary target DNA thanks to the Förster resonance energy transfer, it increases thanks to the 5'-exonuclease activity of the Taq polymerase after hybridization with cleavage of the donor-coupled 5'-terminal nucleotide .
The quantitative real-time PCR using Scorpion primers is also based on the use of Förster resonance energy transfer . Like molecular beacons, these consist of a donor fluorescent dye and quencher-coupled loop-stem structure in combination with a PCR primer . In contrast to other FRET probes, Scorpion primers are incorporated into the synthesized DNA as part of the polymerase chain reaction. As with the use of molecular beacons, a decrease in energy transfer due to an increase in donor fluorescence after excitation as a result of incorporation of the primer and hybridization of the loop-stem structure with the target DNA with dissolution of the tertiary structure can be observed with Scorpion primers.
literature
- Theodor Förster: Intermolecular Energy Migration and Fluorescence , Ann. Physics 6 (2): 55, 1948. doi : 10.1002 / andp.19484370105
- Joseph R. Lakowicz : Principles of Fluorescence Spectroscopy . Plenum Publishing Corporation, 2nd Edition, 1999.
- Entry on Förster-resonance-energy transfer (FRET) . In: IUPAC Compendium of Chemical Terminology (the “Gold Book”) . doi : 10.1351 / goldbook.FT07381 Version: 2.3.1.
Web links
- Olympus: Applications in Confocal Microscopy: Fluorescence Resonance Energy Transfer (FRET) Microscopy. Archived from the original on May 9, 2015 ; accessed on July 13, 2016 .
- Interactive tutorial for optimizing the fluorescence microscopic measurement conditions from the manufacturer Nikon (English)
- FLIM FRET Imaging (tutorial by Becker & Hickl, website)
Individual evidence
- ^ A b S. E. Braslavsky: Glossary of terms used in photochemistry, 3rd edition (IUPAC Recommendations 2006) . In: Pure and Applied Chemistry . tape 79 , no. 3 , January 1, 2007, ISSN 1365-3075 , doi : 10.1351 / pac200779030293 .
- ↑ a b Förster T: Intermolecular energy migration and fluorescence . In: Ann. Physics . 437, 1948, p. 55. doi : 10.1002 / andp.19484370105 .
- ↑ a b Stryer L: Fluorescence energy transfer as a spectroscopic ruler . In: Annu. Rev. Biochem. . 47, 1978, pp. 819-46. doi : 10.1146 / annurev.bi.47.070178.004131 . PMID 354506 .
- ^ Clegg, Robert M .: The history of FRET . In: Lakowicz, Joseph R .; Chris D. Geddes (Ed.): Reviews in Fluorescence 2006 . Springer, Berlin 2006, ISBN 0-387-29342-6 , pp. 1-46.
- ↑ Cario G, Franck J: About the decomposition of hydrogen atoms by excited mercury atoms . In: Z. Physics . 11, 1922, pp. 161-166.
- ↑ Perrin J: Fluorescence et induction molecular par resonance . In: CR Hebd. Seances Acad. Sci. . 184, 1927, pp. 1097-1100.
- ↑ a b Perrin F: Théorie des transferts d'activation entre molécules de méme espèce . In: Ann. Chim. Phys. (Paris) . 17, 1932, pp. 283-314.
- ↑ Kallmann H, London F: About quantum mechanical energy transfers between atomic systems . In: Z. Physics. Chem. B . 2, 1928, pp. 207-243.
- ^ Forster T: Energy migration and fluorescence . In: The natural sciences . 6, 1946, pp. 166-175.
- ↑ a b Marullo S, Bouvier M: Resonance energy transfer approaches in molecular pharmacology and beyond . In: Trends Pharmacol. Sci. . 28, No. 8, August 2007, pp. 362-365. doi : 10.1016 / j.tips.2007.06.007 . PMID 17629577 .
- ↑ a b Demchenko AP: Förster Resonance Energy Transfer . In: Introduction to Fluorescence Sensing . Springer, Berlin 2008, ISBN 1-4020-9002-1 , pp. 91-99.
- ↑ Dexter DL: A theory of sensitized luminescence in solids . In: J Chem Phys . 21, 1953, p. 836. doi : 10.1063 / 1.1699044 .
- ↑ a b c Robert M. Clegg: Forster resonance energy transfer - FRET what is it, why do it, and how it's done . In: Theodorus WJ Gadella (Ed.): FRET and FLIM techniques . Elsevier, Amsterdam 2009, ISBN 0-08-054958-6 , pp. 1-58.
- ↑ Demchenko AP: Förster Resonance Energy Transfer . In: Mechanisms of Signal Transduction . Springer, Berlin 2008, ISBN 1-4020-9002-1 , pp. 249-298.
- ↑ Bazin H, Trinquet E, Mathis G: Time resolved amplification of cryptate emission: a versatile technology to trace biomolecular interactions . In: . Rev. Mol. Biol . 82, No. 3, January 2002, pp. 233-250. doi : 10.1016 / S1389-0352 (01) 00040-X . PMID 11999692 .
- ↑ Mekler VM: A photochemical technique to enhance sensitivity of detection of fluorescence resonance energy transfer . In: Photochem. Photobiol. . 59, August, pp. 615-620. doi : 10.1111 / j.1751-1097.1994.tb09665.x .
- ↑ Laible PD et al. : Detailed Balance in Förster-Dexter Excitation Transfer and Its Application to Photosynthesis . In: J. Phys. Chem. B . 102, No. 9, 1998, pp. 1641-1648. doi : 10.1021 / jp9730104 .
- ↑ Kê, Bacon: photosystem II . In: Photosynthesis: photobiochemistry and photobiophysics . Kluwer Academic, 2001, ISBN 0-7923-6791-X , pp. 199-322.
- ↑ a b Kendall JM, Badminton MN: Aequorea victoria bioluminescence moves into an exciting new era . In: Trends Biotechnol . . 16, No. 5, May 1998, pp. 216-24. PMID 9621461 .
- ↑ Yang X, Neher D: Scherf U, Müllen K (Ed.): Organic Light Emitting Devices: Synthesis, Properties and Applications . Wiley-VCH, Weinheim 2006, ISBN 3-527-31218-8 , pp. 333-368.
- ↑ Kappaun S, Slugovc C, List EJ: Phosphorescent organic light-emitting devices: working principle and iridium based emitter materials . In: Int J Mol Sci . 9, No. 8, August 2008, pp. 1527-1547. doi : 10.3390 / ijms9081527 . PMID 19325819 . PMC 2635741 (free full text).
- ↑ a b Truong K, Ikura M: The use of FRET imaging microscopy to detect protein-protein interactions and protein conformational changes in vivo . In: Curr. Opin. Struct. Biol . 11, No. 5, October 2001, pp. 573-8. PMID 11785758 .
- ↑ Gaits F, Hahn K: Shedding light on cell signaling: interpretation of FRET biosensors . In: Sci. STKE . 2003, No. 165, January 2003, p. PE3. doi : 10.1126 / stke.2003.165.pe3 . PMID 12527820 .
- ↑ Boute N, Jockers R, Issad T: The use of resonance energy transfer in high-throughput screening: BRET versus FRET . In: Trends Pharmacol. Sci. . 23, No. 8, August 2002, pp. 351-354. PMID 12377570 .
- ^ Cooper MA: Advances in membrane receptor screening and analysis . In: J. Mol. Recognit. . 17, No. 4, 2004, pp. 286-315. doi : 10.1002 / jmr.675 . PMID 15227637 .
- ↑ Goddard JP, Reymond JL: Recent advances in enzyme assays . In: Trends Biotechnol. . 22, No. 7, July 2004, pp. 363-370. doi : 10.1016 / j.tibtech.2004.04.005 . PMID 15245909 .
- ↑ Giepmans BN, Adams SR, Ellisman MH, Tsien RY: The fluorescent toolbox for assesing protein location and function . In: Science . 312, No. 5771, April 2006, pp. 217-24. doi : 10.1126 / science.1124618 . PMID 16614209 .
- ↑ Pfleger KD, Eidne KA: Illuminating insights into protein-protein interactions using bioluminescence resonance energy transfer (BRET) . In: Nat. Methods . 3, No. 3, March 2006, pp. 165-74. doi : 10.1038 / nmeth841 . PMID 16489332 .
- ^ Griffin BA, Adams SR, Tsien RY: Specific covalent labeling of recombinant protein molecules inside live cells . In: Science . 281, No. 5374, July 1998, pp. 269-72. PMID 9657724 .
- ↑ Miyawaki A, Griesbeck O, Heim R, Tsien RY: Dynamic and quantitative Ca2 + measurements using improved cameleons . In: Proc. Natl. Acad. Sci. USA . 96, No. 5, March 1999, pp. 2135-40. PMID 10051607 . PMC 26749 (free full text).
- ↑ Ponsioen B, Zhao J, Riedl J, et al. : Detecting cAMP-induced Epac activation by fluorescence resonance energy transfer: Epac as a novel cAMP indicator . In: EMBO Rep. . 5, No. 12, December 2004, pp. 1176-80. doi : 10.1038 / sj.embor.7400290 . PMID 15550931 . PMC 1299185 (free full text).
- ^ Allen JE, McLendon GL: Tryptophan and tyrosine to terbium fluorescence resonance energy transfer as a method to “map” aromatic residues and monitor docking . In: Biochem. Biophys. Res. Commun. . 349, No. 4, November 2006, pp. 1264-8. doi : 10.1016 / j.bbrc.2006.08.165 . PMID 16979582 .
- ↑ a b c Didenko VV: DNA probes using fluorescence resonance energy transfer (FRET): designs and applications . In: BioTechniques . 31, No. 5, November 2001, pp. 1106-16, 1118, 1120-1. PMID 11730017 . PMC 1941713 (free full text).
- ^ Morrison LE, Halder TC, Stols LM: Solution-phase detection of polynucleotides using interacting fluorescent labels and competitive hybridization . In: Anal. Biochem. . 183, No. 2, December 1989, pp. 231-44. PMID 2624314 .
- ^ Tyagi S, Kramer FR: Molecular beacons: probes that fluoresce upon hybridization . In: Nat. Biotechnol. . 14, No. 3, March 1996, pp. 303-8. doi : 10.1038 / nbt0396-303 . PMID 9630890 .
- ↑ Whitcombe D, Theaker J, Guy SP, Brown T, Little S: Detection of PCR products using self-probing amplicons and fluorescence . In: Nat. Biotechnol. . 17, No. 8, August 1999, pp. 804-7. doi : 10.1038 / 11751 . PMID 10429248 .