Star formation
As a star formation is generally referred to those stages of development in the formation of a main sequence star from the collapsing core of a broad molecular cloud are traversed. The initially diffusely distributed interstellar matter condenses by a factor of around 10 18 to 10 20 . Finally, a distinction is made between several collapse phases, namely formation
- of a pre-stellar nucleus,
- of a protostar, and finally
- of a sub-main sequence star.
While low-mass stars can also arise in isolation, the formation of more massive stars mainly takes place in star clusters . These different types of star formation essentially determine the properties and development of galaxies .
Molecular clouds

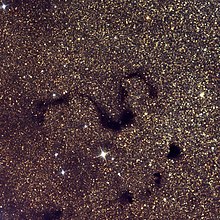
The prerequisite for the formation of stars is the existence of comparatively dense, cold clouds of matter, so-called molecular clouds. The first signs of these clouds emerged from observations in the 18th and 19th centuries: Caroline Herschel reports that her brother Wilhelm Herschel found an apparently starless region, which from today's perspective corresponds to such a molecular cloud, in the constellation Scorpio and with the words " Here is truly a hole in the sky ”commented. It was not until the beginning of the 20th century that large-scale skies were scanned using photographic plates to show that these dark regions were caused by interstellar clouds that obscure the stars behind them. Bart Bok finally identified these dark clouds as the places of star formation, whereas their composition remained a mystery.
Today it is common knowledge that around 70% of these clouds consist of molecular hydrogen (H 2 ) (from which the name “molecular cloud” is derived), which is surrounded by a shell of neutral hydrogen atoms ( HI ). In addition to H 2, there are other molecules in these clouds, such as carbon monoxide (CO). In addition, around 1% of the mass is found in the form of interstellar dust ( silica or graphite particles 0.1 µm in size ).
In the Milky Way , molecular clouds with an average density of around 100 H 2 molecules per cm³ are found mainly in the spiral arms. Some large complexes can diameter of about 150 light years and masses of 10 5 bis 10 6 solar masses (M ☉ ) reach and are therefore referred to as giant molecular clouds (ger .: Giant Molecular Cloud or GMC ). However, there are also smaller, relatively isolated molecular clouds with masses less than a hundred solar masses.
Observation of molecular clouds
Since molecular hydrogen does not have a dipole moment due to its symmetry , it has no observable absorption or emission lines at temperatures of 10–20 K , as is common in molecular clouds, and can therefore not be observed. Instead, indirect observation methods (English tracers ), such as either the presence of proxy molecules or dust, must be used. The most common methods are:
Observations of carbon monoxide
Carbon monoxide (CO) is not only the second most common molecule in such clouds, but has the property that its rotational transitions - transitions from one state of rotation to another, in which infrared light is emitted - can be observed even at low particle densities. The distribution on large scales and the amount of CO molecules follow from such observations. Assuming that the numerical ratio of CO to H 2 molecules is constant, a conversion factor between the H 2 density and the intensity of certain CO spectral lines can be determined; Assuming this conversion factor, the overall structure of the molecular cloud can be reconstructed from measurements on CO and its mass can be determined. Another empirical relationship exists between the extent of the cloud and the line width of the CO lines.
Observations of the wavelength dependence of the absorbance
Blue light is more strongly scattered by the interstellar dust particles than red. This fact can be used to create extinction maps: background stars that shine through a molecular cloud appear systematically redder than their intrinsic color when there is more dust along the line of sight, and less red when there is less dust. The redness is directly proportional to the amount of interstellar dust along the line of sight. Assuming a constant mass ratio of dust to molecular hydrogen, this allows conclusions to be drawn about its distribution and thus about the structure of a molecular cloud. This method is mainly used at near-infrared wavelengths . Here the 2MASS ( Two Micron All-Sky Survey ) with observations at 1.2 µm, 1.6 µm and 2.2 µm has proven to be a real treasure trove for astronomers , as it allows them to create so-called extinction maps of the entire sky.
Far infrared observations
Due to the low temperature of only around 10 K, the thermal emission of the dust particles in the molecular clouds is at wavelengths of around 250 µm. In this wavelength range, molecular clouds are largely optically thin , which allows direct conclusions to be drawn about the amount of dust along the line of sight. However, since this wavelength range is not in an atmosphere window, observations are only possible via satellites, e.g. B. ISO , possible. Launched in 2009, the Herschel satellite telescope offers astronomers unprecedented resolution and sensitivity and has since revolutionized the way astronomers see star formation.
Molecular cloud structure
Clouds | Clumps | Cores | |
---|---|---|---|
Mass (M ☉ ) | 10 3 -10 4 | 50-500 | 0.5-5 |
Expansion ( pc ) | 2-15 | 0.3-3 | 0.03-0.2 |
mean density n (H 2 ) (cm −3 ) | 50-500 | 10 3 -10 4 | 10 4 -10 5 |
Gas temperature (K) | ≈10 | 10-20 | 8-12 |
Simple, analytically solvable star formation models used to be incorrectly based on spherical molecular clouds. This led to the prediction of star formation rates that were significantly too high. For realistic computer simulations of star formation, however, it must be taken into account that molecular clouds have a very pronounced, filamentary structure . Along these filaments, like pearls on a chain, there are condensations that are considered to be the birthplaces of stars. The physical background of this structure is still not fully understood. However, it is assumed that the cause is an interplay of gravitation and turbulence . The turbulence is responsible for the local compressions from which stars form in the further course. Another cause of a local increase in gas density can be the influence of massive stars that push the material together and compress it through star winds.
A hierarchical structure is usually defined in a molecular cloud. Although this division probably has no physical background and a molecular cloud more of a fractal has structure, the division into is cloud (Engl. Cloud ,) lump (Engl. Clump and) core (Engl. Core ) spread common and far. The entire structure is referred to as the cloud , a clump is a physically coherent subgroup and a core is a gravitationally bound unit that is usually seen as the direct predecessor of a protostar.
First collapse
Molecular cloud nuclei collapse

Stars are formed from dense molecular cloud cores that are embedded in a molecular cloud. A wide variety of forces act within such a molecular cloud. The most important thing is gravity , which through its attractive effect ensures that these nuclei contract further. This collapse is mainly counteracted by the thermal energy , i.e. the proper movement of the molecules, which they only have due to their temperature. However, magnetic fields and / or turbulence can also be important for stability .
Stability and collapse
The so-called virial analysis provides a simple means of stability analysis . If a molecular cloud core is in equilibrium, the kinetic energy of the particles and their potential gravitational energy just balance out (neglecting magnetic fields and turbulence). In the event that the gravitational energy predominates, the collapse of this core inevitably follows. The virial analysis is easy to perform for a nucleus with a homogeneous density, but only serves as a rough estimate of the stability of a molecular cloud. For a real cloud to be stable, the pressure inside must be higher than in layers further out. If this is taken into account in the stability analysis, the criterion for stability is a density ratio between the center and the shell. In the borderline case for critical stability, one speaks of a Bonnor-Ebert sphere, and the stability criterion can be converted into a so-called Bonnor-Ebert mass , which the cloud must exceed for a collapse to occur.
If a molecular cloud core exceeds its critical mass (i.e. the thermal movement of the particles cannot counteract its own gravity), collapse inevitably follows. As soon as the limit of instability is exceeded, the contraction takes place almost in free fall, i.e. the layers falling inward feel only the gravitational potential and fall unchecked (and thus in particular faster than the local speed of sound ) towards the center. The collapse spreads from the inside to the outside at the speed of sound ("Inside-Out Collapse"): The region that collapses becomes larger and larger around the densest core regions, and more and more of the previously static, thin gas is included in the collapse included.
As mentioned, a core initially has an increased density in the center, which is why the collapse occurs faster in this region than in the shell. During this collapse, gravitational energy is converted into thermal energy and emitted in the mm wavelength range . However, since the outer shells are permeable to radiation of these wavelengths, the gravitational binding energy is completely radiated outwards. This is why this first phase is isothermal , so the temperature of the core does not change at first.
If magnetic fields play a role, the situation becomes much more complicated. Electrons and ions move helically around the magnetic field lines. A collapse is possible unhindered in their direction. The physics is more complicated for movements perpendicular to the field lines. By binding the ionized matter to the magnetic field, it hinders the collapse, but vice versa is also carried along by the matter. As matter thickens, so does the magnetic field. Neutral and ionized gas couple to each other through collisions of molecules and ions and move together. With increasing density, the degree of ionization falls and the coupling becomes weaker, i.e. This means that the time scale on which matter can diffuse both charges across the field lines into denser areas ( ambipolar diffusion ) is decreasing . However, the available time also decreases in free fall, and the magnetic voltage increases as the field lines deform.
In the further course of the collapse, the density increases further, the shell becomes optically thick for the radiation and thus causes heating. This heating slowly leads to the formation of a hydrostatic equilibrium in the center, which slows down the collapse and finally stops.
This so-called first core , also known as First Hydrostatic Core (FHSC), which largely consists of hydrogen molecules, has a radius of typically 10 to 20 AU , which is roughly three times the radius of Jupiter's orbit . In the center, the collapse is now stopped for the time being, but the areas of the shell lying further outside continue to fall in free fall onto this first core. The impact of the matter on the hydrostatic core leads to the formation of shock waves , which finally heat up the core even more. This first phase of star formation from collapse to the formation of a hydrostatic core takes around 10,000 years and is defined by the so-called free fall time .
Observation of pre-stellar nuclei
Prestellar nuclei are observed using the same methods as molecular clouds. On the one hand, the dust they contain absorbs the light from background stars, which is why they can be seen as star-free areas in the optical and near infrared. On the other hand, they also radiate due to their temperatures of around 10 K at (sub) mm wavelengths and can be seen there through the thermal emission of the dust.
Just like molecular clouds in general, pre-stellar nuclei are also observed and detected with the help of molecular lines . In contrast to the molecular cloud, which is mainly detected by CO, various effects are used when observing nuclei. On the one hand, the center of a core is protected from the interstellar radiation field by its shell. This creates chemical compounds there that would be destroyed by this radiation. This means that there are molecules in pre-stellar nuclei that cannot be found in the interstellar medium. On the other hand, the nuclei are so dense that these molecules are stimulated to higher states by collisions with hydrogen molecules and emit characteristic spectral lines.
The chemistry within such a pre-stellar core is still the subject of current research, since in addition to chemical reactions in the gas phase, the so-called freezing of molecules on dust particles and the associated chemistry of the dust particles must also be taken into account.
The transition phase from a pre-stellar to a protostellar nucleus, i.e. the observation of a first nucleus , is also unknown and has never been observed before . Some candidates for such an object have been discovered so far, but no confirmed observation has been made to date.
Protostars

Second collapse
The so-called first nucleus only heats up until the temperature is sufficient to split the hydrogen molecules into their individual atoms . However, the energy that is used is no longer available to stabilize the core. This leads to a second collapse, which is only stopped when a hydrostatic equilibrium is established again. The second nucleus, however, consists mainly of hydrogen atoms and is about one and a half solar radii . A pre-stellar nucleus has finally become a protostar : a star that is still gaining in mass and derives its luminosity primarily from accretion from outside onto the object of falling matter.
Although this protostar already radiates at a temperature of a few 1000 K, it is covered from the outside by the dense shell surrounding it. Its radiation, however, gradually heats up the molecular cloud from within. In the inner regions the temperature rises to over 1500 K, so that all hot dust particles evaporate. A largely radiation-permeable region (“opacity gap”) forms there inside the dust jacket.
If the temperature in the central areas of the molecular cloud rises above temperatures of around 100 K, the molecules begin to evaporate from the ice shell around the dust particles and pass into the gas phase. In this so-called Hot Corino , a large number of chemical reactions take place due to the increased temperatures and high frequency of molecules in the gas phase . The processes in these regions are thus in contrast to those in the cold outer areas of the protostellar cloud, which in terms of their conditions still resemble the pre-stellar nuclei.
The outer hull areas, which are still in free fall , continue to rain down on the protostars and thus ensure a steady increase in mass. Most of the luminosity is obtained from this accretion process. However, only around 1% of the total mass of the molecular cloud core is still in the central star. The phase in which the star steadily increases in mass due to the incidence of shell material is called the main accretion phase . In a simplified view, this collapse takes place radially symmetrically. On closer inspection, however, the molecular cloud nuclei have an angular momentum other than zero , so that dust and gas cannot easily fall onto the central star.
Circumstellar disk and jets
A collapse requires a redistribution of the angular momentum. This often leads to the formation of double or multiple star systems , or perpendicular to the axis of rotation of a circumstellar disk . An effective transport of angular momentum is possible within this disk, which on the one hand leads to the fact that particles migrate towards the central star, but on the other hand also to an expansion of the disk, as the particles that absorb angular momentum drift further outwards. This disk can have an area of around 100 AU.
In addition to a circumstellar disk, these protostars form bipolar, strongly collimated jets perpendicular to it . These are created through an interplay of rotation, magnetic fields and accretion. It is believed that FHSCs (first nuclei) can already form weak molecular outflows, while jets are formed in the later evolutionary phase. These are fed by material from the circumstellar disk. They bump into the surrounding shell material at supersonic speed, which leads to the formation of shocks. These shocks are very hot, and this enables chemical reactions that can lead to the formation of new molecules. In addition to the jets with speeds of a few 100 km / s, there are also slower, less collimated outflows of molecular matter with speeds of up to a few 10 km / s. This is probably a material that the jet pulls with it when flowing through the envelope. The jet slowly eats a cavity in the protostellar cloud. This cavity is initially still very narrow, with opening angles of only a few degrees, but continues to expand as time progresses and ensures the dispersion and thinning of the envelope in the direction of the outflows.
The protostar itself continues to accrete matter. However, it no longer falls directly and isotropically on it, but is mainly recorded via the circumstellar disk (which is why it is often also called the accretion disk ).
Classification of protostars
Spectral class | Spectral index |
---|---|
Class 0 | - |
Class I. | α> 0.3 |
Flat spectrum | 0.3> α> −0.3 |
Class II | −0.3> α> −1.6 |
Class III | α <−1.6 |
For a more precise evolutionary classification serving astronomers called here spectral energy distributions (Engl. Spectral energy distribution , SED), the color index and in particular the so-called spectral index
.
Here is the wavelength and the flux density . Usually, the spectral index for near infrared wavelengths between 2.2 and 10 μm is used for classification. The radiation from protostellar systems is dominated by thermal radiation. In the early phase with temperatures of a few 10 K, the radiation maximum is far in the far infrared and the radiation intensity therefore increases with increasing wavelength (α> 0). When reaching the main sequence, the SED is dominated by the central star with temperatures of a few 1000 K with the radiation maximum in the optical and a resulting negative spectral index.
Observation of protostars
In the optical and near-infrared wavelength range, protostellar nuclei hardly differ from pre-stellar nuclei. The dense shell swallows the light from the stars behind, which is why they are also recognizable as dark regions in the sky. At (sub) mm wavelengths you can also see the thermal radiation of the dust in the envelope.
Differences can be seen when observing the wavelengths in between, since the envelope becomes transparent at these wavelengths. As these observations in the middle and far infrared were not possible from the earth's surface due to the atmosphere, this gap could only be closed with the help of satellite missions and advancing detector technology.
The most important instrument for observing these early phases of star formation was the IRAS satellite mission , which systematically examined the entire sky with broadband filters with central wavelengths of 12 µm, 25 µm, 60 µm and 100 µm. Class 0 protostars could mostly only be detected in the longer wavelengths, depending on the distance to the object, as they are still too cold to radiate strongly at wavelengths of only a few 10 µm. With the start of the Spitzer Space Telescope in 2003, however, due to its higher sensitivity, even at shorter wavelengths (e.g. 24 μm), a number of class 0 protostars could be discovered in molecular clouds that had previously been thought to be starless. These objects form the new class of so-called VeLLOs (Very Low Luminosity Objects) and are the subject of current research.
In addition to the thermal radiation of the protostellar cloud, it is also possible to observe the bipolar outflow of matter. For this purpose one often observes molecular line transitions of CO (and its isotopes ). They allow conclusions to be drawn about velocities in the outflows or about excitation conditions (density, temperature, ...). Other molecules, e.g. B. can only be formed in the extreme environmental conditions of jets are often used to study the nature of jets. The rotation signature of the disk can also be seen with the help of various molecular line transitions. However, the small size of these disks makes spatial resolution difficult, which is why interferometric recordings are often necessary.
Spectral classification
Stars are characterized and classified during their formation phase using spectral energy distribution (SED). The shape of the SED of class 0 protostars is similar to that of a cold black body with a temperature of only around 20–30 K. A protostar has already formed in the center; however, its radiation is completely absorbed by the tight cover and ensures that it is heated up.
The SED of class I protostars is still dominated by the thermal radiation from the cold dust envelope. However, at shorter wavelengths, the black body radiation of the protostar appears in the center, which already has a temperature of a few 1000 K. In addition to the thermal radiation, the SED also shows the spectral characteristics of the shell material. The radiation maximum at 10 µm is due to dust in the form of silicates .
Sub-sequence stars
In the early phase of star formation, the protostar draws a large part of its luminosity from accretion of material from the shell. In the further course of evolution, however, this decreases more and more, and the luminosity is mainly supplied by the self-contraction of the central star. At this stage one no longer speaks of a protostar, but of a pre-main sequence star.
T-Tauri stars and Herbig-Ae / Be stars
The astronomical nomenclature for stars at this stage is based on their mass: With a mass of less than 2 solar masses one speaks of T-Tauri stars , for more massive stars with up to 8 solar masses of Herbig-Ae / Be stars .
In the case of T-Tauri stars, the shell is already thinned so far that it allows a direct view of the central star and the surrounding disk. It turns out that these young stars are largely covered by “ star spots ” as a result of strong magnetic fields . Furthermore, T-Tauri stars have strong winds , so that further accretion, around 10 −9 to 10 −7 solar masses per year, only happens via the protoplanetary disk , which initially accounts for around 0.5% of the mass of the central star. Over the course of around 2 million years, the disk dissolves through various processes (accretion, jets , photoevaporation and others).
If T-Tauri stars still have strong emission lines at the beginning , their intensity decreases as the protoplanetary disk dissolves. This is why one speaks of Weak T-Tauri Stars (WTTS) in contrast to the classic T-Tauri Stars (English classical T-Tauri Star CTTS). As the gas mass in the disk decreases, so does the jet activity.
In the Hertzsprung-Russell diagram (HRD), T-Tauri stars appear above the main sequence and initially move almost vertically down the Hayashi line . The temperature increases in the center, but initially it is only sufficient for the energetically insignificant fusion of primordial deuterium and lithium . First of all, the star is optically thick, so that the gravitational energy released inside reaches the outside by convection . Stars with a mass of more than 0.5 solar masses sooner or later form a compact core zone, the high acceleration of which prevents convection. With the restriction to radiation transport, the temperature rises, also in the outer, convective part of the envelope, and the pre-main sequence star swings in the HRD on an almost horizontal evolutionary path. Finally, the nuclear fusion of hydrogen sets in and prevents further contraction, the star has reached the main sequence. Stars with a mass of less than 0.5 solar masses remain fully convective until they reach the main sequence. Stars with less than 0.08 solar masses (about 80 Jupiter masses ) do not reach the core temperature necessary for hydrogen burning. Their contraction ends when the electrons degenerate . As a result, these “failed stars” cool down as brown dwarfs .
Observation of pre-main sequence stars
Pre-main sequence stars can be observed using the same methods as protostars. Furthermore, there is also the possibility of observing the protoplanetary disk through scattered light. The physics of the scattering processes allows conclusions to be drawn about the type of scattering dust particles.
With new telescopes (e.g. ALMA ) it will also be possible in the future to directly observe gaps in protoplanetary disks caused by planets. Indirect evidence of this has already been found in spectra at infrared wavelengths. In young main sequence stars and pre-main sequence stars of class III, in which the gas in the disk has almost completely evaporated and so-called debris disks are left, it is possible to observe planets directly.
Spectral classification
The spectral energy distribution of T-Tauri stars is dominated by the black body radiation of the central star. The protoplanetary disk, however, creates an excess of radiation in the mid and far infrared. Due to the different components of the pane with different temperatures, this excess radiation cannot be described by a black body with a single temperature.
With the slow thinning of the protoplanetary disk, its radiation component disappears almost completely and the radiation of the pre-main sequence star remains. In some systems, however, there is still a small excess of radiation that usually indicates debris disks.
Star formation in clusters
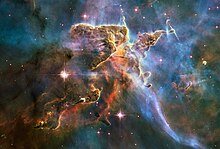
While stars with low mass can also be formed in isolation, more massive stars only form in so-called clusters, which arise from larger molecular clouds. In such star clusters , various processes can lead to modifications of the classical paradigm of star formation. Two protostars that accret material from the same molecular cloud core compete with one another and can stop the mass influx on the other protostar; Jets and outflows can invade other protostellar systems, and tidal forces can act as an additional disruptive factor. These are just a few examples of how star formation in isolation can differ from formation processes in clusters.
Another factor that distinguishes these two schemes of star formation (isolation vs. cluster) from one another is the appearance of more massive stars in clusters. In contrast to the formation of stars like our sun , which is completed after around 10 million years, massive stars with masses of more than 8 solar masses form in a much shorter time. In principle, the same evolutionary stages are passed through (gravitational collapse, formation of a disk and jets), but not so strongly differentiated over time. While the nuclear fusion processes are already beginning in the center , the star is still covered by a dense dust cover. This mainly affects the observability of massive pre-main sequence stars, which can therefore mainly be observed at infrared and longer wavelengths.
Since more massive stars have higher surface temperatures of several 10,000 K, their thermal radiation consists mainly of UV and soft X-rays . The radiation pressure can become so great that it prevents further accretion. Furthermore, this radiation is able to ionize neutral hydrogen atoms in the shell . For O stars , this so-called H-II region can have a diameter of around 100 light years. The ionization and the subsequent recombination lead to the emission of the hydrogen series, the dominant line being the Hα line of the Balmer series with 656.3 nm.
Just as quickly as these massive stars formed, their nuclear fuel is depleted; the stars eventually end up as supernovae . In the process, elements created explosively by nuclear fusion are released into the interstellar medium. Pressure waves emanating from them can lead to local condensation of the surrounding molecular cloud, which then becomes gravitationally unstable and in turn forms new stars.
Star populations
The preceding sections describe the best-understood star formation processes: star formation in today's universe. In the early days of cosmic history, however, the conditions were significantly different, and this requires other models of star formation: When stars reach the main sequence, they get their energy almost exclusively from nuclear fusion processes. This ultimately leads to the formation of helium , carbon and other heavier elements, including iron . These elements finally reach the interstellar medium via star winds or through huge supernova explosions and enrich it with metals (whereby in astronomy all elements apart from hydrogen and helium are commonly referred to as metals). These metals play a very important role in star formation. Dust particles, like some molecules (e.g. CO), ensure efficient cooling of molecular cloud cores, which ultimately leads to gravitational instability and thus to collapse.
However, stars in the early universe could only form from the light elements that formed shortly after the Big Bang . Therefore, the star formation processes must differ fundamentally from our understanding of star formation today. One possible mechanism is the mass formation of hundreds to millions of stars in clusters, in which tidal forces and complex interactions between the cluster members play an important role. The metal-poor stars that are formed, so-called Population III stars , are likely to have become much heavier and thus also hotter than stars today.
The successor generation of stars, the so-called star population II, already had an accumulation of metals in the astronomical sense - elements heavier than helium - which did not reach the abundance ratios of stars like our sun (which belongs to the so-called population I), but already a significant one allowed faster cooling of the molecular clouds concerned, so that stars could form preferentially, the mass of which is smaller than that of our sun. While Population III stars have not yet been observed, the halo of our Milky Way, an area with a relatively low star formation rate, contains population II stars that are poor in metal. In the disk of the Milky Way itself, however, there are mainly Population I stars.
While first generation Population III stars have not yet been discovered, the oldest star in Population II to date was discovered in 2014, with an age of around 13.6 billion years ( SMSS J031300.36-670839.3 , SM0313).
Star formation in galaxies
Star formation is a key process in the formation and evolution of galaxies . The central question is where and how efficiently gas is converted into stars in galaxies.
Galaxies can be divided into those that are still forming new stars on a larger scale and those in which star-forming activity has largely come to a standstill. This classification corresponds to a characteristic color distribution of the galaxies with a group of bluish (active star formation) and a group of reddish (hardly star formation) galaxies. The development trends of these two galaxy types are a key observation of the galaxy development: The number of star-forming galaxies remains largely the same per considered ( expanding ) cosmic volume, while the number of "dead" galaxies has increased steadily over the last 10 billion years.
As early as the 1970s it was recognized that deformed galaxies - according to today's understanding the results of the interaction of several galaxies with one another - have a bluish color than conventional galaxies of the same type. A comparison with models showed that the properties of such galaxies indicate comparatively short phases of intense star formation, namely only a few tens of millions of years. Such galaxies are called (also in German) starburst galaxies .
In our home galaxy, the Milky Way , around one solar mass of new stars is created every year.
literature
- EA Bergin, M. Tafalla: Cold Dark Clouds: The Initial Conditions for Star Formation. In: Annual Review of Astronomy & Astrophysics. Vol. 45, 2007, pp. 339-396 ( bibcode : 2007ARA & A..45..339B , arxiv : 0705.3765 ).
- J. Blum, G. Wurm: The Growth Mechanisms of Macroscopic Bodies in Protoplanetary Disks. In: Annual Review of Astronomy & Astrophysics. Vol. 46, 2008, pp. 21-56 ( bibcode : 2008ARA & A..46 ... 21B ).
- V. Bromm, RB Larson: The First Stars. In: Annual Review of Astronomy & Astrophysics. Vol. 42, 2004, pp. 79–118 ( bibcode : 2004ARA & A..42 ... 79B , arxiv : astro-ph / 0311019 ).
- Bradley W. Carroll, Dale A. Ostlie: An Introduction to Modern Astrophysics. 2nd edition. Pearson, 2007, ISBN 0-321-44284-9 .
- Bradley W. Carroll, Dale A. Ostlie: An Introduction to Modern Galactic Astrophysics and Cosmology. 2nd edition. Pearson, 2007, ISBN 0-8053-0347-2 .
- EF van Dishoeck: ISO Spectroscopy of Gas and Dust: From Molecular Clouds to Protoplanetary Disks. In: Annual Review of Astronomy & Astrophysics. Vol. 42, 2004, pp. 119-167 ( bibcode : 2004ARA & A..42..119V , arxiv : astro-ph / 0403061 ).
- CP Dullemond, JD Monnier: The Inner Regions of Protoplanetary Disks. In: Annual Review of Astronomy & Astrophysics. Vol. 48, 2010, pp. 205-239 ( bibcode : 2010ARA & A..48..205D , arxiv : 1006.3485 ).
- CF McKee, EC Ostriker: Theory of Star Formation. In: Annual Review of Astronomy & Astrophysics. Vol. 45, 2007, pp. 565-687 ( bibcode : 2007ARA & A..45..565M , arxiv : 0707.3514 ).
- Steven W. Stahler, Francesco Palla: The Formation of Stars. Wiley-VCH, Weinheim 2004, ISBN 3-527-40559-3 .
- Albrecht Unsöld, Bodo Baschek: The New Cosmos: Introduction to Astronomy and Astrophysics. 7th edition. Springer, 2005, ISBN 3-540-42177-7 .
- JP Williams, LA Cieza: Protoplanetary Disks and Their Evolution. In: Annual Review of Astronomy & Astrophysics. Vol. 49, 2011, pp. 67–117 ( bibcode : 2011ARA & A..49 ... 67W , arxiv : 1103.0556 ).
- Helmut Zimmermann, Alfred Weigert: ABC Lexicon Astronomy. 9th edition. Spectrum Academic Publishing House, Heidelberg 1999.
- H. Zinnecker, HW Yorke: Toward Understanding Massive Star Formation. In: Annual Review of Astronomy & Astrophysics. 2007, Vol. 45, pp. 481-563 ( bibcode : 2007ARA & A..45..481Z , arxiv : 0707.1279 ).
Individual evidence
- ^ HE Houghton: Sir William Herschel's 'Hole in the Sky'. In: Monthly Notes of the Astronomical Society of South Africa. Vol. 1, pp. 107-108.
- ^ EE Barnard : Catalog of 349 Dark Objects in the Sky. University of Chicago Press, Chicago 1927, ( bibcode : 1927cdos.book ..... B ).
- ^ BJ Bok, EF Reilly: Small Dark Nebulae. In: Astrophysical Journal . Vol. 105, 1947, pp. 255-257 ( bibcode : 1947ApJ ... 105..255B ).
- ^ JS Mathis, W. Rumpl, KH Nordsieck: The size distribution of interstellar grains. In: Astrophysical Journal . Vol. 217, 1977, pp. 425-433 ( bibcode : 1977ApJ ... 217..425M ).
- ^ JP Williams, L. Blitz, CF McKee: The Structure and Evolution of Molecular Clouds: from Clumps to Cores to the IMF. In: V. Mannings, AP Boss, SS Russell (eds.): Protostars and Planets IV. University of Arizona Press, Tucson 2000, pp. 97–120. ( Bibcode : 2000prpl.conf ... 97W , arxiv : astro- ph / 9902246 ).
- ↑ E.g. Dan P. Clemens, Richard Barvainis: A catalog of small, optically selected molecular clouds - Optical, infrared, and millimeter properties. In: Astrophysical Journal Supplement Series. Volume 68, 1988, pp. 257-286 ( bibcode : 1988ApJS ... 68..257C ).
- ↑ Section 2.1.2. in Bergin & Tafalla 2007; Conversion factor AW Strong, JR Mattox: Gradient model analysis of EGRET diffuse Galactic γ-ray emission. In: Astronomy and Astrophysics . Volume 308, pp. L21-L24 ( bibcode : 1996A & A ... 308L..21S ).
- ↑ PM Solomon, AR Rivolo, J. Barrett, A. Yahil: Mass, luminosity, and linewidth relations of Galactic molecular clouds. In: Astrophysical Journal. Vol. 319 (1987), pp. 730-741 ( bibcode : 1987ApJ ... 319..730S ).
- ^ CJ Lada, EA Lada, DP Clemens, J. Bally: Mapping Dust Extinction With IR Cameras. In: Ian S. McLean (Ed.): Infrared Astronomy with Arrays: The Next Generation. Astrophysics and Space Science Library, Vol. 190, 1994, ISBN 0-7923-2778-0 , pp. 17 ff. ( Bibcode : 1994ASSL..190..473L ).
- ↑ M. Lombardi, J. Alves: Mapping the interstellar dust with near-infrared observations: An optimized multi-band technique. In: Astronomy and Astrophysics. Vol. 377, 2001, pp. 1023-1034 ( bibcode : 2001A & A ... 377.1023L , arxiv : astro-ph / 0109135 ).
- ↑ Dirk Froebrich, Jonathan Rowles: The structure of molecular clouds - II. Column density and mass distributions. In: Monthly Notices of the Royal Astronomical Society. Vol. 406 (2010), pp. 1350-1357 ( bibcode : 2010MNRAS.406.1350F , arxiv : 1004.0117 ).
- ^ Z. BEF van Dishoeck 2004.
- ↑ Ph. André u. a .: From filamentary clouds to prestellar cores to the stellar IMF: Initial highlights from the Herschel Gould Belt Survey. In: Astronomy and Astrophysics. Vol. 518, id. L102 ( bibcode : 2010A & A ... 518L.102A , arxiv : 1005.2618 ).
- ^ EA Bergin, M. Tafalla: Cold Dark Clouds: The Initial Conditions for Star Formation. In: Annual Review of Astronomy & Astrophysics. Vol. 45 (2007), pp. 339-396 ( bibcode : 2007ARA & A..45..339B , arxiv : 0705.3765 ).
- ↑ A. Men'shchikov et al. a .: Filamentary structures and compact objects in the Aquila and Polaris clouds observed by Herschel. In: Astronomy and Astrophysics. Vol. 518, id L103 ( bibcode : 2010A & A ... 518L.103M , arxiv : 1005.3115 ).
- ↑ a b Mordecai-Mark Mac Low, Ralf S. Klessen: Control of star formation by supersonic turbulence. In: Reviews of Modern Physics. Vol. 76 (1) (2004), pp. 125-194 ( bibcode : 2004RvMP ... 76..125M , arxiv : astro-ph / 0301093 ).
- ↑ Section 2.6., In particular Table 1, in Bergin & Tafalla 2007.
- ↑ Section 2.2. in McKee & Ostriker 2007, Section 3.2 in Stahler & Palla 2004.
- ↑ R. Ebert: About the compression of H I areas. In: Journal of Astrophysics. Vol. 37 (1955), pp. 217-232 ( bibcode : 1955ZA ..... 37..217E )
- ^ WB Bonnor: Boyle's Law and Gravitational Instability. In: Monthly Notices of the Royal Astronomical Society. Vol. 116, pp. 351-359 ( bibcode : 1956MNRAS.116..351B ). See p. 35 f. in Bergin & Tafalla 2007.
- ↑ p. 59 f. in McKee & Ostriker 2007; Section 11.1.2 in Stahler & Palla 2004.
- ↑ Section 10.2. in Stahler & Palla 2004.
- ↑ Section 10.3. in Stahler & Palla 2004.
- ↑ p. 31 f. in Stahler & Palla 2004.
- ↑ Dunham et al. a .: Detection Of A Bipolar Molecular Outflow Driven By A Candidate First Hydrostatic Core . In: ApJ . tape 742 , no. 1 , 2011, doi : 10.1088 / 0004-637X / 742/1/1 ( iop.org (PDF)).
- ^ Richard B. Larson: Numerical calculations of the dynamics of collapsing proto-star . In: mnras . tape 145 , 1969, bibcode : 1969MNRAS.145..271L .
- ↑ Sections 11.1.2. and 11.1.3. in Stahler & Palla 2004.
- ↑ M. Nielbock, R. Launhardt, J. Steinacker, AM Stutz, Z. Balog: The Earliest Phases of Star formation (EPoS) observed with Herschel: the dust temperature and density distributions of B68 . In: Astronomy & Astrophysics . tape 547 , November 2012, ISSN 0004-6361 , p. A11 , doi : 10.1051 / 0004-6361 / 201219139 ( aanda.org [accessed August 23, 2019]).
- ^ R. Launhardt, AM Stutz, A. Schmiedeke, Th. Henning, O. Krause: The Earliest Phases of Star Formation (EPoS): a Herschel key project: The thermal structure of low-mass molecular cloud cores ⋆⋆⋆⋆⋆ ⋆ . In: Astronomy & Astrophysics . tape 551 , 2013, ISSN 0004-6361 , p. A98 , doi : 10.1051 / 0004-6361 / 201220477 ( aanda.org [accessed August 23, 2019]).
- ↑ ML Enoch, J.-E. Lee, P. Harvey, MM Dunham, and S. Schnee: A Candidate Detection of the First Hydrostatic Core. In: The Astrophysical Journal Letters. Vol. 722 (2010), pp. L33-L38 ( bibcode : 2010ApJ ... 722L..33E , arxiv : 1009.0536 ).
- ↑ a b Section 11.1.2 in Stahler & Palla 2004.
- ↑ Section 11.1.3 in Stahler & Palla 2004.
- ↑ S. Bottinelli et al. a .: Complex Molecules in the Hot Core of the Low-Mass Protostar NGC 1333 IRAS 4A. In: Astrophysical Journal. Vol. 615 (2004), pp. 354-358 ( bibcode : 2004ApJ ... 615..354B , arxiv : astro-ph / 0407154 ).
- ↑ Section 12 in Stahler & Palla 2004.
- ↑ Section 11.3. in Stahler & Palla 2004.
- ↑ Section 13 in Stahler & Palla 2004.
- ↑ Figure 15 in MN Machida et al .: High- and Low-Velocity Magnetized Outflows in the Star Formation Process in a Gravitationally Collapsing Cloud. In: Astrophysical Journal. Vol. 676 (2008), pp. 1088-1108 ( bibcode : 2008ApJ ... 676.1088M ).
- ↑ Section 13.5 in Stahler & Palla 2004.
- ↑ BA Wilking et al. a .: Infrared Properties of Weak Radio Sources in the ρ Ophiuchi Molecular Cloud. In: The Astrophysical Journal. Vol. 551 (2001), pp. 357-366 ( bibcode : 2001ApJ ... 551..357W ).
- ↑ J. di Francesco et al. a .: An Observational Perspective of Low-Mass Dense Cores I: Internal Physical and Chemical Properties. In: B. Reipurth, D. Jewitt, K. Keil (eds.), Pp. 17-32 ( bibcode : 2007prpl.conf ... 17D , arxiv : astro-ph / 0602379 ). Tucson: University of Arizona Press 2007.
- ↑ p. 611 in Stahler & Palla 2004.
- ↑ p. 61 f. in Stahler & Palla 2004.
- ↑ E. Gullbring, Eric L. Hartmann, C. Briceno, N. Calvet: Disk Accretion Council for T Tauri stars. In: Astrophysical Journal. Vol. 492 (1998), p. 323 ( bibcode : 1998ApJ ... 492..323G ).
- ↑ For example, E. Mamajek: Initial Conditions of Planet Formation: Lifetimes of Primordial discs. In: Exoplanets and Disks: Their Formation and Diversity: Proceedings of the International Conference. AIP Conference Proceedings, Vol. 1158 (2009), pp. 3-10 ( bibcode : 2009AIPC.1158 .... 3M , arxiv : 0906.5011 ).
- ↑ p. 98 in Stahler & Palla 2004.
- ↑ E.g. David Lafrenière, Ray Jayawardhana, Marten H. van Kerkwijk: The Directly Imaged Planet Around the Young Solar Analog 1RXS J160929.1 - 210524: Confirmation of Common Proper Motion, Temperature, and Mass. In: Astrophysical Journal. Vol. 719 (2010), pp. 497-504 ( bibcode : 2010ApJ ... 719..497L , arxiv : 1006.3070 ).
- ↑ Section 17.3.1 in Stahler & Palla 2004.
- ↑ Section 15.5.5 in Stahler & Palla 2004.
- ↑ See sections 3.1. and 3.2. in Bromm & Larson 2004.
- ↑ Section 3.5. in Bromm & Larson 2004.
- ↑ Sebastian Anthony: We've found the oldest star in the known universe. 12th of February 2014.
- ^ For example, Eric F. Bell et al. a .: Nearly 5000 Distant Early-Type Galaxies in COMBO-17: A Red Sequence and Its Evolution since z ~ 1. In: Astrophysical Journal. Vol. 608, 2004, pp. 752-767 ( bibcode : 2004ApJ ... 608..752B , arxiv : astro-ph / 0303394 ).
- ↑ Iskra Strateva et al. a .: Color Separation of Galaxy Types in the Sloan Digital Sky Survey Imaging Data. In: Astronomical Journal. Vol. 122, 2001, pp. 1861–1874 ( bibcode : 2001AJ .... 122.1861S , arxiv : astro-ph / 0107201 )
- ↑ Gabriel B. Brammer et al. a .: The Number Density and Mass Density of Star-forming and Quiescent Galaxies at 0.4 ≤ z ≤ 2.2. In: Astrophysical Journal. Vol. 739, 2011, article number 24 ( bibcode : 2011ApJ ... 739 ... 24B , arxiv : 1104.2595 ).
- ^ First Richard B. Larson, Beatrice M. Tinsley: Star formation rates in normal and peculiar galaxies. In: Astrophysical Journal. Vol. 219, 1978, pp. 46-59. Bradley W. Carroll, Dale A. Ostlie: An Introduction to Modern Galactic Astrophysics and Cosmology. 2nd edition. Pearson, 2007, ISBN 0-8053-0347-2 , pp. 50 f.
- ^ Thomas P. Robitaille, Barbara A. Whitney: The Present-Day Star Formation Rate of the Milky Way Determined from Spitzer-Detected Young Stellar Objects. In: Astrophysical Journal Letters. Vol. 710 (2010), pp. L11-L15 ( bibcode : 2010ApJ ... 710L..11R , arxiv : 1001.3672 ).