Supernova
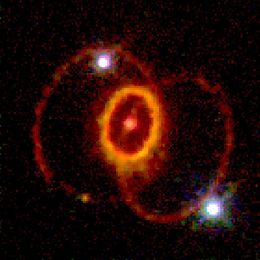
A supernova (from Latin stella nova, super , 'new star, beyond' ; plural supernovae ) is the brief, bright flashing of a massive star at the end of its life through an explosion, in which the original star itself is destroyed. The star's luminosity increases millions to billions of times, for a short time it becomes as bright as a whole galaxy .
About one Foe of observable energy is released within seconds . This corresponds to a value of ≈ 3 · 10 28 TWh ( Tera watt hours ). For comparison: if the sun had its current luminosity during its entire lifespan, it would release 3.827 · 10 26 W × 3.1536 · 10 7 s / year × 10 10 years ≈ 1.2 foe of energy.
Two basic mechanisms are known by which stars can become supernovae:
- Massive stars with an initial mass (see star formation ) of more than about eight solar masses , the core of which collapses at the end of their evolution and after their nuclear fuel has been used up. A compact object such as a neutron star ( pulsar ) or a black hole can arise here. This process is known as a collapse or hydrodynamic supernova .
- Star lower mass, which in its preliminary-stage as white dwarf material (. For example by an attendant in a binary system ) accrete , by self-gravity to collapse and thereby by incipient carbon-burning process will be torn. This phenomenon is known as a thermonuclear supernova or type Ia supernova .
Well-known supernovae are the Supernova 1987A in the Large Magellanic Cloud and Kepler's Supernova (1604) . The latter in particular and Tycho Brahe's Supernova (1572) have given astronomy wings, as it has finally refuted the classical view of the immutability of the fixed star sphere . The most famous supernova remnant is the Crab Nebula ( Supernova 1054 ) in the constellation Taurus .
year | observed in | maximum apparent brightness |
---|---|---|
185 | Centaur constellation | −6 m |
386 | Sagittarius constellation | +1.5 m |
393 | Scorpio constellation | −3 m |
1006 | Constellation wolf | −7.5 ± 0.4 m |
1054 | Taurus constellation | −6 m |
1181 | Cassiopeia constellation | −2 m |
1572 | Cassiopeia constellation | −4 m |
1604 | Serpent Bearer Constellation | −2 m |
1680 | Cassiopeia constellation | +6 m |
1885 | Andromeda Nebula | +6 m |
1979 | Galaxy Messier 100 | +11.6 m |
1987 | Large Magellanic Cloud | +3 m |
2014 | Galaxy Messier 82 | +10.5 m |
history
The name of the nova goes back to the name Tycho Brahe coined for an observation of a star in 1572 . It refers to the sudden appearance of a previously invisible star-like object in the firmament . Up to the middle of the 20th century, a nova was understood to mean any kind of outbreak of brightness in a star with an increase to its maximum over a period of days to years and a return to its earlier brightness within weeks or decades (see light curve ). When the astrophysical cause of the eruptions was recognized, the term changed to the current definition, in which a supernova is no longer one of the novas in its original meaning.
At the beginning of the 20th century there was still no explanation for the appearance of new or temporary stars , as supernovae were then called. There were several hypotheses , including one by Hugo von Seeliger , according to which the entry of a solid body into a cosmic cloud of finely distributed matter (with which one imagined space was filled) leads to a strong heating of the surface of this body and thus to a glow . The observed shifts in the spectrum of the new stars were interpreted as an indication that the formation of their dense shell must have taken place in a few days.
designation
Supernovae are named with the prefix “SN”, their year of discovery and an alphabetical addition. Originally, this addition consisted of a capital letter that was assigned alphabetically in the order of discovery. Such was SN 1987A , the first discovered in 1987 supernova. In 1954 (in distant galaxies) more than 26 supernovae were discovered in one year for the first time. Since then, small double letters (from “aa” to “zz”) have been used from the 27th supernova of a year. With modern large telescopes and special search programs , several hundred supernovae were discovered per year in the 2000s: in 2005 there were 367 (up to SN 2005nc), in 2006 there were 551 (up to SN 2006ue), and in 2007 even 572 (up to SN2007uz). Today there are well over a thousand per year.
frequency
How often supernovae appear in a galaxy depends on how many stars are newly formed in it. This is because very massive stars that end in supernovae only have a short lifespan of tens of millions of years according to astronomical time standards. About 20 ± 8 supernovae per millennium are estimated for the Milky Way , of which six were observed in the last millennium. About two thirds of the galactic supernovae remained hidden by the extinction of the galactic disk; the rest of the observed supernovae were found in other galaxies.
In the Milky Way, the last, even were freiäugig visible supernovae in 1572 by Brahe and in 1604 by Kepler observed. A very distant one followed in 1680 , but was only visible through a telescope . For modern astrophysics , however, the SN 1885A in the Andromeda Galaxy and especially the one from 1987 in the relatively nearby Large Magellanic Cloud became significant .
classification
SN I | SN II | ||||
---|---|---|---|---|---|
early spectrum does not contain any hydrogen lines |
early spectrum contains hydrogen lines | ||||
SN Ia | SN Ib | SN Ic | SN IIb | SN II-L | SN II-P |
Spectrum contains silicon |
no silicon | Helium line dominant |
Hydrogen lines dominate | ||
a lot of helium |
little helium |
Light goes back linearly
to the maximum |
Light stays at a high level for
a while after maximum |
Historically, there are roughly two types of supernovae. The classification is based on the criterion whether or not spectral lines of hydrogen are visible in their light in the early stages of the supernova . On the one hand there is type I, in which no hydrogen lines are visible, with the subgroups Ia, Ib and Ic; and on the other hand type II with hydrogen lines (see table). The rough type designations were introduced by Rudolph Minkowski in 1939 and have been refined since then.
This division into Type I and Type II does not coincide with the two physical mechanisms mentioned in the introduction that can lead to a supernova. Rather, only supernovae of subtype Ia are thermonuclear.
Thermonuclear supernovae of type Ia
A type Ia supernova is created according to a common model in cataclysmic binary star systems consisting of a white dwarf and a companion. Over time, the white dwarf accretes gas from the extensive shell of its companion, which can lead to several nova outbreaks. During these outbreaks, the hydrogen of the accreted gas fuses, the fusion products remain until the core of the white dwarf in front of the supernova contains large amounts of oxygen-contaminated carbon, comparable to a giant diamond . The average density prevailing under high gravitational pressure is typically around 3 t per cm³. As the nucleus approaches the Chandrahsekharmasse through further accretion and combustion processes in the shells , it becomes increasingly unstable. The more mass it is fed, the smaller its radius, the density increases to over 1000 t per cm³. According to Pauldrach, in this state it is more of a border crosser than a star that no longer has a specifiable radius. When the limit mass is reached, the carbon does not ignite due to an increase in temperature, but due to the further increase in density. The degenerate star only perceives the resulting increase in temperature when it reaches a normal thermal, non-degenerate state again at around 10 billion K. The entire carbon supply is burned to iron and nickel in a fraction of a second and the star can react normally to the scenario again, i.e. that is, it explodes in a thermonuclear supernova of type Ia.
A second route to cross the Chandrasekhar boundary can be the super soft X-ray sources . Here the mass transfer rate to the white dwarf is high enough to result in permanent hydrogen burning .
However, this standard model came under pressure due to observations made by the Chandra X-ray telescope. Measurements on six selected galaxies showed that the soft X-ray radiation is 50 times lower than the value to be expected if Novae and Super Soft X-ray Sources were the dominant sources for Supernova-Ia explosions. Since then there has also been speculation about other forerunners:
- a binary star system in which a white dwarf accretes gas from the shell of a red giant
- two white dwarfs revolving around and finally merging
- the central star of a planetary nebula
The second explanatory model is also known as the “two-fold degenerate scenario”. A close binary star system of white dwarfs begins to exchange matter (so-called AM-Canum-Venaticorum stars ). Either one of the stars crosses the Chandrasekhar limit (as with the cataclysmic double stars), or the supernova explosion is caused by a merger of the two white dwarfs.
However, there are not enough of the first two scenarios to explain the number of Type Ia supernovae observed and - in the case of merging white dwarfs - over-Chandrasekharmasse supernovae would be expected.
The starting point in the third scenario are red giants on the asymptotic giant branch of sufficient mass. After the outer shell (the later planetary nebula) has been pushed off, these central stars develop into white dwarfs with a mass above the Chandrasekhar limit, which burn hydrogen and helium in their outer shells over a core of carbon and oxygen. As soon as the combustion processes in the shells have produced enough carbon and deposited it on the compact core so that it exceeds the limit mass, the carbon in the core ignites and the star explodes in a type Ia supernova.
According to different theoretical models, nuclear fusion can take place both as detonation and as deflagration . According to recent work, which is hotly debated among experts, the most likely scenario is an initial deflagration that turns into a detonation. Other theories speak of magnetic fields from which the explosion energy is taken.
The released energy of such a supernova explosion lies within defined limits, since the range of the critical mass and the composition of white dwarfs is known. Because of this property, it is called a standard candle and is suitable for determining distances (see below).
In a type Ia supernova explosion, no compact object is left - all of the matter of the white dwarf is hurled into space as a supernova remnant . The companion star becomes a so-called "runaway" star, as it flies away at the - normally high - orbital velocity with which it has orbited its partner star so far.
Core collapse or hydrodynamic supernova
Forerunner star
According to the now generally accepted theory of gravitational collapse , which was first proposed by Fritz Zwicky in 1938 , a supernova of this type occurs at the end of the “life” of a massive star when it has completely used up its nuclear fuel . Stars with initial masses of around 8 to 10 to around 30 solar masses end their existence as stars in a type II explosion, stars with greater mass explode as type Ib / c. Type Ib or Ic supernovae go through a Wolf-Rayet stellar phase before the explosion , in which they repel their outer, still hydrogen-rich layers in the form of a stellar wind .
In the case of a roughly spherically symmetrical star structure , the following sequence results: As soon as the hydrogen in the core of the star is fused to form helium ( hydrogen burning ), the internal pressure of the star generated by the fusion energy drops and the star then collapses under the influence of its gravity. This increases the temperature and density , and another stage of fusion begins , the three-alpha process , in which helium fuses to carbon via the intermediate product beryllium ( helium burning ). The process (nuclear fuel exhaustion, contraction, next fusion stage) is repeated, and carbon burning creates neon . Further fusion stages ( neon burning , oxygen burning and silicon burning ) allow the shrinking star to fuse ever new elements. If a fuel in the core of the star has dried up, the fusion reaction changes the spherical shell lying above the core and continues there as shell burning , while the fusion product in the shrinking core becomes the new "fuel". However, each fusion stage releases less energy than its predecessor and runs faster. While a massive star with about eight solar masses spends tens of millions of years in the hydrogen burning stage, the subsequent helium burning takes “only” a few million years, the carbon burning only about 50,000 years. The last fusion stage of silicon firing can be measured in hours to days. Because each previous fusion stage also lasts longer in the shell burning than the subsequent fusion stages in the star, the star develops a kind of onion structure with several fusing shells: In the last stage silicon burns in the core and oxygen, neon, carbon in the layers above. , Helium and hydrogen burning take place (in Wolf-Rayet stars , however, the hydrogen shell is missing, and sometimes also the helium). Due to the extremely short duration of the fusion steps after carbon burning, the last fusion steps have practically no influence on the externally visible stellar parameters - the energy generated inside does not come to the surface until the final collapse. This is also the reason why supernovae can apparently take place without any warning at any supergiant that appears to be outwardly normal (i.e. there is neither an abnormal change in luminosity nor a change in diameter, temperature, spectrum, etc.). The "fusion bulb" present in the center of the dying supergiant is tiny in relation to the star's diameter.
During their long lifetimes, all of these stars go through the various energy- releasing fusion chains in their core up to the synthesis of iron , the element with atomic number 26. This is where the fusion chain ends, since iron atomic nuclei have the highest binding energy per nucleon of all atomic nuclei. Fusions to heavier elements require external energy and do not release any more.
The speed at which a star converts the fuel in its interior depends on the temperature and the density and thus indirectly on the gravitational pressure on its core. An important consequence of this relationship is that a star consists of layers in which the transposition speed decreases towards the outside. Even if the helium burning has already started in the core , hydrogen burning still takes place in the layers above. The absolute rate of fusion in the core increases with stellar mass strongly to. While a star with a solar mass needs about 10 billion years to go through the fusion chain in its core until it comes to a standstill, the lifespan of extremely heavy stars with about 100 solar masses is only on the order of a few million years. See late stages of stellar evolution for a more detailed overview.
Core collapse

a) developed layers of elements, iron core in the center
b) iron core begins to collapse, black arrows: outer layers at supersonic speed, white arrows: inner core at subsonic speed
c) conversion of the core into neutrons, radiation of the binding energy in the form of Neutrinos
d) incident matter is reflected on the core, red: resulting shock wave running outwards
e) energy conversion in nuclear processes, shock wave runs out, neutrinos accelerate mass again
f) outer matter is ejected, degenerate residue remains
The iron, the "ashes" of nuclear burning, remains in the core of the star. As soon as no more fusions take place, all radiation ends, which counteracted gravitation with its outward pressure and inflated the star. Two further processes intensify this effect: First, the photons of high-energy gamma radiation destroy iron atomic nuclei by means of photo-disintegration . This creates α-particles and neutrons ; the α-particles in turn can be broken down into their core components , protons and neutrons, by such photons . Due to the high stability of iron cores, this process requires energy. Second, in the so-called inverse β-decay ( electron capture ), free electrons are captured by protons. In the process, more neutrons are created and neutrinos are released (Jerry Cooperstein and Edward A. Baron, 1990). Both the energy loss due to the photo-disintegration and the loss of free electrons cause a further reduction in the external pressure counteracting gravity.
Now gravity can have its full effect. Eventually the core crosses the Chandrasekhar boundary and collapses.
The collapse of the central area happens so quickly - within milliseconds - that the speed of incidence already exceeds the local speed of sound of the medium at a distance of 20 to 50 km from the center . The inner layers can only transport the print information quickly enough because of their high density. The outer layers fall into the center as a shock wave . As soon as the inner part of the nucleus reaches densities at the nuclear level, it already consists almost entirely of neutrons because the electrons are pressed into the protons (reversal of the beta decay ). Neutron collections also have an upper limit mass ( Tolman-Oppenheimer-Volkoff limit , depending on the model about 2.7 to 3 solar masses), above which a black hole occurs. Here the mass is now lower in order to consider the other case. The core becomes incompressible due to quantum mechanical rules ( degeneracy pressure ), and the collapse is stopped almost suddenly. This causes a gigantic increase in pressure and density in the center, so that even the neutrinos can no longer escape unhindered. This pressure information is reflected on the neutron nucleus and now runs out again. The pressure wave quickly reaches areas with too low a speed of sound that are still in the incidence. Another shock wave is created, but it is now moving outwards. The material traversed by the shock front is very strongly compressed, as a result of which it reaches very high temperatures (Bethe, 1990). A large part of the energy is consumed by further photo-disintegration when passing through the outer iron core. Since the nuclear binding energy of all iron is roughly equal to the energy of the shock wave, it would not break out of the star and not cause an explosion without a renewal. As a correction, the neutrinos are also considered as an additional source of energy and momentum . Normally, neutrinos hardly interact with matter. However, there are so high neutrino densities in the collision front that the interaction of the neutrinos with the matter there can no longer be neglected. Since the vast majority of the total energy of the supernova goes into the neutrinos, a relatively low absorption is sufficient to rekindle the shock and break it out of the collapsing iron core. After leaving the iron core, when the temperature has dropped enough, the pressure wave gains additional energy through renewed fusion reactions.
The extremely strongly heated gas layers, which drag the neutron-rich material with them from the outer areas of the central area, incubate heavy elements beyond iron in the so-called r process ( r from rapid, "fast"), such as copper , germanium , Silver , gold or uranium . About half of the elements on planets beyond iron originate from such supernova explosions, while the other half was spawned in the s process by stars with less mass and released into space in their giant phase .
The heated gas masses expand rapidly behind the shock front. The gas gains outward speed. A few hours after the collapse of the central area, the surface of the star is reached and the gas masses are blasted off in the now visible supernova explosion. The shell of the supernova reaches speeds of millions of kilometers per hour. In addition to the energy released as radiation, the majority of 99% of the energy released during the collapse is released in the form of neutrinos. They leave the star immediately after the density of the initially impenetrable collision front has become sufficiently small. Since they move almost at the speed of light , they can be measured by terrestrial detectors a few hours before the optical supernova, such as supernova 1987A .
Another “early warning signal” for the lighting up of a core collapse supernova is a so-called X - ray burst . This occurs when the waves of the shock front reach the star's surface and break out into the interstellar medium - days before the outbreak of brightness is observed in visible light. Such an X-ray signal was observed for the first time in January 2008 with NASA's Swift satellite during the 2008D Supernova .
Supernovae, with the exception of type Ia, are also referred to as hydrodynamic supernovae because they are caused by the collapse of the central area. The scenario presented is based on the widespread consensus in science that supernova explosions of massive stars in principle proceed in this way. However, there is still no complete and functioning physical model of a supernova explosion that all scientists involved in it agree with.
Supernova types II-L and II-P
Supernovae of type II, are distinguished by the criterion whether the brightness of the Super Nova with time rather l decreases inear (type SN II-L), or during the decay of a P lateauphase passes (type SN II-P). The peak values of the absolute brightness show a wide spread with SN II-P , while most SN II-L have almost the same maximum brightness. The average brightness in the blue spectral range of SN II-P reaches −17.0 mag with a standard deviation of 1.1 mag, while SN II-L is mostly −17.6 ± 0.4 mag. The existence of plateau phases is explained by the fact that the ejected mass and thus the speed of the supernova's envelope is very large. The decrease in brightness due to cooling is compensated for by the rapid expansion of the shell due to the increased surface area and the light curve is described by a plateau. The maximum brightness depends on the radius of the previous star, which explains the large spread in the maximum brightnesses of the SN II-P. Type II-L supernovae have a slower expansion rate, so that their brightness is determined by radioactive processes in the early stages. As a result, there is less scattering of the maximum brightnesses (Young, Branch, 1989). The Supernova SN 1979C is an example of the Type II-L. Here, however, only the brightness in visible light decreased; In the X-ray range , the supernova is just as bright today as it was when it was discovered in 1979. The mechanism that causes this persistent brightness has not yet been fully explored.
Supernova types Ib and Ic
In the case of type Ib supernovae, the hydrogen envelope was repelled before the explosion, so that no spectral lines of the hydrogen can be observed during the explosion. The explosion type Ic occurs when the helium shell of the star has also been repelled so that no spectral lines of the helium appear. These explosions are also caused by a core collapse and a compact object remains.
A similar spectral course to type Ib - but less bright - occurs with a calcium rich gap transient supernova .
Supernova remnants
The material ejected by the supernova forms an emission nebula , the so-called “ supernova remnant ”, in contrast to the remnant of the core collapse that may arise, which is referred to as a “compact object” in astrophysics . Probably the most famous supernova remnant is the Crab Nebula , which was ejected when the SN1054 exploded . This supernova also left a compact object (a pulsar ) behind.
Compact objects
The shape of the remnant that remains from the star depends on its mass. Not all of the outer layers are thrown away in the supernova explosion. The remaining gas accretes onto the collapsed core in the center, which consists almost entirely of neutrons. The falling gas is also broken down into neutrons by the processes described above, so that a neutron star is created. If the star becomes even heavier (more than about 3 solar masses) due to the falling material, the gravitational force can also overcome the counter pressure caused by the Pauli principle , which separates the neutrons from each other in a neutron star and thereby stabilizes it (see degenerate matter ). The remnants of the star finally collapse and form a black hole from whose gravity field no more signals can escape. More recent observations suggest that there is another intermediate form, the so-called quark stars , whose matter is made up of pure quarks .
Due to the pirouette effect, neutron stars often rotate at a very high speed of up to 1000 revolutions per second; this already follows from the conservation of angular momentum during collapse.
The high speed of rotation generates a magnetic field that interacts with the particles of the ejected gas nebula and therefore generates signals that can also be recorded from Earth . In the case of neutron stars, one speaks of pulsars.
Pair instability supernova
A variant of the core collapse scenario is to pair instability supernova (pair instability supernova, PISN), does not collapse when the star into a compact object, but is completely torn. The forerunner stars are particularly poor in elements that are heavier than helium. The pressure in the core is not high enough to be able to form heavy elements such as iron, which is the prerequisite for core collapse. In this phase, after the end of the helium burning, the star reaches temperature and density ranges in which the photon energies lead to the generation of electron - positron pairs. This leads to a reduction in the radiation pressure and thus to a further rapid increase in the density - and thus the temperature - of the core, until the oxygen and silicon burning begins to explode, which builds up a new counter pressure against the gravitational pressure. Depending on the magnitude of the gravitational pressure - and thus the mass of the core - this core explosion can slow down or even prevent further collapse. In the case of a PISN, there is no compact remnant, but the star is completely torn apart. The energies released are up to 100 foe (10 46 J ) by about a factor of 100 above those of a "normal" core collapse supernova.
Model calculations for vanishing metallicity and without taking into account a possible rotation or magnetic fields provide a critical mass of the helium nucleus of 64 solar masses for the onset of pair instability. If the mass of the helium nucleus is greater than 133 solar masses, the nuclear explosion cannot prevent further collapse, which therefore continues to develop into a black hole. If these helium nuclear masses are extrapolated to the necessary total mass of a main sequence star (neglecting mass losses), the PISN has a mass range of around 140 to 260 solar masses. Because of this, this scenario is considered extremely rare in today's universe. It is mainly considered for the first generation of stars of the so-called Population III . There, however, this mechanism could have played an important role in the enrichment of the intergalactic medium with heavier elements.

The supernova SN 2006gy in the galaxy NGC 1260 is a special case , which was discovered on September 18, 2006 as part of the Texas Supernova Search : The absolute brightness of SN 2006gy was more than one magnitude higher than that of other supernovae. The discoverers therefore interpret this supernova, which is about 240 million light years away, as the first candidate for which the pair instability mechanism is possible as an explanation - however, neither the previous data nor the theoretical models are sufficient to be able to make a clear decision here.
The first reliable representative of a PISN is the supernova SN 2007bi , which was discovered on April 6, 2007 in a dwarf galaxy in the constellation Virgo. A group of astronomers from the Weizmann Institute for Sciences used the two Keck telescopes , among other things , to observe the spectra and the course of brightness for more than a year. The investigations showed that the precursor star of the remnant of the stars, 1.7 billion light years away, was an unusually massive and metal-poor hypergiant with probably 200 solar masses. In an unusually slow process, large amounts of silicon and radioactive nickel were also released.
Distance measurements with the help of supernovae
Since the radiation, especially in the later course of a type Ia supernova, is largely fed by the radioactive decay of 56 Ni to 56 Co and from this to 56 Fe , the half-lives being about 6 and 77 days respectively (this theory was first proposed by Fred Hoyle and William Alfred Fowler in 1960), the shape of the light curve is always approximately the same. Due to the mechanism, the amount of energy released should also always be approximately the same, which, due to the approximately identical structure, always results in approximately the same luminosity. Due to these properties of a standard candle, relatively precise distance measurements in space can be made using such supernova explosions . The time scale of the light curve can also be used to determine the redshift in addition to the spectral lines , since a redshift of z. B. 2, the timing for the observer is extended by this factor. The idea for this goes back to Fritz Zwicky . By measuring the distance of supernova explosions, which occurred about 7 billion years ago, one can prove the accelerated expansion of the universe (see e.g. Hubble constant or Supernova Cosmology Project ). In order to really use supernovae as standard candles, however, the mechanisms of explosion must be better researched and understood.
Computer simulations of supernovae
Stirling Colgate and Richard White carried out the first hydrodynamic numerical calculations on supernovae at the Lawrence Livermore National Laboratory in 1966 and recognized the importance of neutrinos for the explosion mechanism. James R. Wilson made other important advances in the early 1980s. Other well-known scientists who have worked on supernova simulations are W. David Arnett , Stanford E. Woosley , Wolfgang Hillebrandt and Fiona Harrison .
More recent calculations (as of 2016) that work with methods similar to those that have proven themselves in the calculation of flame turbulence in gasoline engines and based on the most advanced description of the crucial neutrino physics in collapsing stars without forced symmetry assumptions, provide results that represent an important milestone for supernova modeling . They confirm the fundamental possibility that neutrino heating triggers the explosion of massive stars. As already seen with the earlier two-dimensional (i.e. rotationally symmetrical) models, non-radial flow processes support the onset of the explosion and imprint asymmetries on the expanding matter, which lead to the asymmetries observed later in supernovae.
The assumption that many massive stars end up either very faintly or completely without an explosion in a so-called un-nova (as also assumed with the core collapse of the predecessor of Cygnus X-1 ) and thus do not explode visibly, can, however, due to the enormous computing time required not yet proven in a simulation.
The increasingly faster supercomputers made it possible to carry out supernova calculations without unnatural assumptions about symmetry. This made simulations much more realistic, since the relevant physics is taken into account in the models, especially with regard to the highly complex interactions of neutrinos , such simulations are at the absolute limit of what is currently feasible on the largest available supercomputers.
In 2016, 16,000 processor cores were made available to a team at the Max Planck Institute for Astrophysics (MPA) on the SuperMUC at the Leibniz Computing Center (LRZ) in Garching and on the MareNostrum at the Barcelona Supercomputing Center (BSC). Even if these 16,000 processor cores are used in parallel, a single model simulation of a supernova still takes six months over a development time of around 0.5 seconds and consumes around 50 million hours of computing time.
Impact on the earth
The possible outbreak of a supernova near the solar system is known as a near-earth supernova . It is assumed that distances to the supernova of less than 100 light years would have noticeable effects on the Earth's biosphere . The gamma rays from such a supernova can trigger chemical reactions in the upper layers of the atmosphere, during which nitrogen is converted into nitrogen oxides . This could completely destroy the ozone layer , exposing the earth to dangerous radiation.
The mass extinction in the Upper Ordovician , in which about 50 percent of the oceanic species became extinct, is associated by some authors with such a near-Earth supernova. Some researchers suspect that a past near-Earth supernova can still be detected through traces of certain metal isotopes in rock layers. Enrichments of the isotope 60 Fe were found, for example, in deep-sea rocks of the Pacific Ocean .
The most potentially dangerous supernovae are Type Ia . Since they arise from a narrow, semi-separated binary star system consisting of a faint accreting white dwarf and a companion losing mass, cataclysmic variables appear rather inconspicuous and it is conceivable that precursors of such a supernova remain undiscovered or are only insufficiently studied in relative proximity to Earth. Some predictions suggest that such a supernova could still affect Earth at distances of up to 3000 light years. The nearest known candidate for a future supernova of this type is IK Pegasi , about 150 light years away.
Type II supernovae, on the other hand, are considered less dangerous. More recent studies (from 2003) assume that such a supernova must light up at a distance of less than 26 light years in order to double the biologically effective UV radiation on earth.
Others
In October 2011, the Nobel Committee awarded the three American astrophysicists Saul Perlmutter , Brian Schmidt and Adam Riess the Nobel Prize in Physics for their observations on supernovae . In the 1990s - contrary to the prevailing doctrine - they found out that dark energy is driving the universe apart at increasing speed.
The SN 2016aps discovered in 2016 was classified as the most luminous supernova to date (as of April 2020) .
See also
- List of supernovae
- nuclear astrophysics
- Hypernova
- Interaction-powered supernova
- Supernova Cosmology Project
- Iax-type supernova
- Electron capture supernova
- Bright red nova
literature
- DH Clark, FR Stephenson: The Historical Supernovae. Pergamon Press, Oxford et al. a., 1977, ISBN 0-08-020914-9 .
- J. Cooperstein, E. Baron: Supernovae: The Direct Mechanism and the Equation of State. In: Supernovae. Edited by A. G. Petschek, Springer 1990.
- H. Bethe : Supernova mechanisms. Reviews of Modern Physics, Vol. 62, No. October 4, 1990.
- Wolfgang Hillebrandt , H.-T. Janka, Ewald Müller: Puzzling Supernova Explosions. Spectrum of Science, edition 7/2005, p. 36 ff.
- Richard F. Stephenson et al. a .: Historical supernovae and their remnants. Clarendon Press Oxford 2004, ISBN 0-19-850766-6 .
- Wolfgang Hillebrandt, Bruno Leibundgut (Eds.): From twilight to highlight - the physics of supernovae. Springer, Berlin 2003, ISBN 3-540-00483-1 .
- Gerald North: Observing variable stars, novae, and supernovae. Cambridge Univ. Press, Cambridge 2004, ISBN 0-521-82047-2 .
- Peter Höflich u. a .: Cosmic explosions in three dimensions - asymmetries in supernovae and gamma-ray bursts. Cambridge Univ. Press, Cambridge 2004, ISBN 0-521-84286-7 .
Web links
- Dramatic compression of matter in collapsing stars changes the shape of atomic nuclei . ( Memento from December 8, 2008 in the Internet Archive ) In: Wissenschaft.de.
- Astronomers are finding the remains of the oldest historically recorded supernova observed by Chinese astronomers in AD 185 . ( Memento from December 8, 2008 in the Internet Archive ) In: Wissenschaft.de.
- Record - researchers observe brightest supernova monster star explosion . In: Wissenschaft.de.
- The super supernova star explosion was as bright as a hundred billion suns . ( Memento from October 12, 2007 in the Internet Archive ) In: Wissenschaft.de.
- Supernova Cosmology Project . In: supernova.lbl.gov. Retrieved February 23, 2012.
- The Secret of the Brightest Starbursts In: astronews.com. 16th February 2017.
Spektrum.de: The secret of particularly strong supernovae February 5, 2019
Videos
- What is a supernova? from the alpha-Centauri television series(approx. 15 minutes). First broadcast on Oct. 24, 1999.
- What if a supernova explodes? From the alpha-Centauri TV series(approx. 15 minutes). First broadcast on April 27, 2005.
- Supernova companion star system . An artistic NASA / ESA simulation of the supernova SN 1993J, a spectacular type Ia supernova from 1993 (available in various Quick-Time and MPEG formats).
Individual evidence
- ^ Hartmann DH: Afterglows from the largest explosions in the universe . In: Proc. Natl. Acad. Sci. USA . 96, No. 9, April 1999, pp. 4752-5. bibcode : 1999PNAS ... 96.4752H . doi : 10.1073 / pnas.96.9.4752 . PMID 10220364 . PMC 33568 (free full text).
- ^ Patrick Moore: The Data Book of Astronomy. CRC Press, 2000, ISBN 978-1-4200-3344-1 .
- ^ P. Frank Winkler, G. Gupta: The SN 1006 Reminant: Optical Proper Motions, Deep Imaging, Distance, and Brightness at Maximum . In: The Astrophysical Journal . 585, 2003, pp. 324-335. doi : 10.1086 / 345985 .
- ↑ Tycho Brahe. In: The Brockhaus Astronomy. Mannheim 2006, p. 63.
- ^ Meyer's Large Conversational Lexicon . 6th edition. Bibliographisches Institut, Leipzig / Vienna 1909 ( zeno.org [accessed on October 17, 2019] lexicon entry “Fixed stars”).
- ↑ TNS Transients Statistics and skymaps. In: Avishay Gal-Yam, International Astronomical Union, Division D Working Group Supernovae. 2019, accessed October 23, 2019 .
- ↑ Adalbert WA Pauldrach: The Dark Universe. The Dark Matter-Dark Energy Contest: Was the Universe Born to Die? , 2nd edition, Springer 2017 ( ISBN 978-3-662-52915-7 ), page 379ff.
- ^ Walter Lewin, Michael van der Klies: Compact Stellar X-ray Sources (Cambridge Astrophysics) . Cambridge University Press, Cambridge 2010, ISBN 978-0-521-15806-0 .
- ↑ Adalbert WA Pauldrach: The Dark Universe. The Dark Matter-Dark Energy Contest: Was the Universe Born to Die? , 2nd edition, Springer 2017 ( ISBN 978-3-662-52915-7 ), pages 420ff.
- ↑ Adalbert WA Pauldrach: The Dark Universe. The Dark Matter-Dark Energy Contest: Was the Universe Born to Die? , 2nd edition, Springer 2017 ( ISBN 978-3-662-52915-7 ), pages 426ff.
- ↑ Gamezo, Khokhlov, Oran, 2004.
- ↑ Hans-Thomas Janka: Supernovae and cosmic gamma-ray bursts. ISBN 978-3-8274-2072-5 , p. 74.
- ↑ In Search of the Cosmic Origin of Silver . In: uni-heidelberg.de.
- ↑ Camilla J. Hansen et al. a .: Silver and palladium help unveil the nature of a second r-process. A&A, Vol. 545, id. A31, September 2012, bibcode : 2012A & A ... 545A..31H .
- ^ Roger Chevalier: Astronomy: Supernova bursts onto the scene. Nature 453, 462-463 (May 22, 2008), doi: 10.1038 / 453462a .
- ↑ D. Richardson, D. Branch, D. Casebeer, J. Millard, RC Thomas, E. Baron: A Comparative Study of the Absolute Magnitude Distributions of Supernovae . In: The Astronomical Journal . 123, No. 2, 2002, pp. 745-752. bibcode : 2002AJ .... 123..745R . doi : 10.1086 / 338318 .
- ^ A b Heger, Woosley, Baraffe, Abel: Evolution and Explosion of Very Massive Primordial Stars, Lighthouses of the Universe: The Most Luminous Celestial Objects and Their Use for Cosmology: Proceedings of the MPA / ESO / MPE / USM Joint Astronomy Conference Held in Garching. Germany, 6. – 10. August 2001, ESO Astrophysics Symposia, ISBN 3-540-43769-X . Edited by M. Gilfanov, R. Sunyaev, and E. Churazov. Springer-Verlag, 2002, p. 369 arxiv : astro-ph / 0112059 .
- ↑ First pair instability supernova discovered . In: Astronomie-heute.de. December 3, 2009.
- ↑ a b Melson, Tobias; Janka, Hans-Thomas: Computer simulations show successful star explosions in three dimensions . In: Max Planck Institute for Astrophysics, Garching, Stellar Astrophysics . 2016. doi : 10.17617 / 1.1N .
- ↑ A. Melott et al. a .: Did a gamma-ray burst initiate the late Ordovician mass extinction? . In: International Journal of Astrobiology . 3, No. 2, 2004, pp. 55-61. arxiv : astro-ph / 0309415 . doi : 10.1017 / S1473550404001910 .
- ↑ Researchers Detect 'Near Miss' Supernova Explosion , University of Illinois College of Liberal Arts and Sciences . Fall / Winter 2005–2006, p. 17. Archived from the original on April 27, 2007. Retrieved on February 1, 2007.
- ↑ K. Knie et al. a .: 60 Fe Anomaly in a Deep-Sea Manganese Crust and Implications for a Nearby Supernova Source . In: Physical Review Letters . 93, No. 17, 2004, pp. 171103-171106. doi : 10.1103 / PhysRevLett.93.171103 .
- ^ BD Fields, J. Ellis: On Deep-Ocean Fe-60 as a Fossil of a Near-Earth Supernova . In: New Astronomy . 4, 1999, pp. 419-430. arxiv : astro-ph / 9811457 . doi : 10.1016 / S1384-1076 (99) 00034-2 .
- ↑ Michael Richmond: Will a Nearby Supernova Endanger Life on Earth? . April 8, 2005. Archived from the original on March 6, 2007. Retrieved March 30, 2006. See Section 4.
- ↑ Mark Gorelick: The Supernova Menace . In: Sky & Telescope . March 2007.
- ↑ Neil Gehrels, Claude M. Laird et al. a .: Ozone Depletion from Nearby Supernovae . In: Astrophysical Journal , Volume 585, March 10, 2003, pp. 1169-1176, doi: 10.1086 / 346127 , arxiv : astro-ph / 0211361
- ↑ Supernova - younger, faster, further . In: tagesspiegel.de. October 5, 2011.