Nuclear explosion
Nuclear weapon explosions (also atomic explosions , nuclear explosions ) are the most powerful human-made explosions to date . They are triggered by the ignition of nuclear weapons . In the simplest case of a nuclear fission bomb , a nuclear chain reaction starts immediately after the critical mass is exceeded . In an air explosion, in addition to the typical explosion cloud , fireball , pressure wave and radioactive residues arise in the atmosphere. The first man-made atomic explosion occurred on July 16, 1945 in an area known as the White Sands Missile Range in the New Mexico desert . By nuclear explosions at nuclear tests increase in induced radioactivity in the Earth's atmosphere led in 1963 to the Treaty Banning Nuclear Weapon Tests in the Atmosphere, in Outer Space and Under Water . Since then, test explosions by the contracting parties have always taken place underground. The US atomic bombs on Hiroshima and Nagasaki on August 6 and 9, 1945 in the war against Japan at the end of World War II were the first and so far only nuclear weapons deployments. The possibility of nuclear war has been an issue since the early days of the Cold War . In the course of the arms race between the superpowers USA and Soviet Union and the other nuclear powers, it developed into a threat to the continued existence of mankind.
introduction
The most noticeable difference to conventional explosions is the much larger amount of energy and the high temperatures. In nuclear explosions, temperatures of over 100 million Kelvin are reached, whereas chemical explosions only have temperatures of up to a few thousand Kelvin. The high temperature of atomic bomb explosions is also the reason for the formation of the characteristic, brightly glowing fireball. The explosive effect of an atomic explosion is usually specified in the unit of kilotons or megatons of TNT equivalent , which relates the explosion energy to the chemical explosive TNT .
The description of a nuclear explosion on the basis of its explosive power is misleading compared to conventional explosions insofar as, in addition to the strong pressure wave , an atomic bomb explosion affects its environment primarily through intense heat radiation from the fireball (including visible light ) as well as through ionizing direct radiation and radioactive residues ( fallout ). The latter in particular make nuclear explosions dangerous, since their effect is not limited to the moment of the explosion, but can last for many years. Electrical and electronic systems are usually affected or destroyed by a strong nuclear electromagnetic pulse (NEMP) in the event of explosions at low or particularly high altitudes .
The physical process as well as the militarily intended effect of nuclear weapon explosions was examined in numerous atom bomb tests by the USA and the Soviet Union , especially in the 1950s . Most of the physical knowledge of the explosion and its impact on the environment came from such experiments, while the medical, economic, and social consequences of the atomic bombs dropped on Hiroshima and Nagasaki in August 1945 were studied. Some of this information has now been released for publication.
Types of explosion
In connection with atomic explosions, one often speaks of detonations . Physically, however, this is not correct, because a detonation requires a reaction front that propagates through the energy-releasing medium at supersonic speed. In nuclear fission, however, there is no reaction front, and nuclear fusion inside a hydrogen bomb is more like deflagration . Only the chemical explosive that compresses the fissile material into a supercritical mass detonates.
The simple form of a ground explosion, i.e. igniting nuclear weapons directly at the target, is not the most effective. Atomic explosions develop their greatest destructive power when ignited in the air; from a tactical point of view, such an application is usually more effective. Ground and subsurface explosions are only more effective in a few special cases - for example as bunker breakers .
Air explosions
Air explosions are those within the lower layers of the atmosphere (below 30 kilometers) where the fireball does not touch the ground. The pressure wave of the explosion spreads like a soap bubble and is first reflected in the hypocenter (earth's surface under the bomb, ground zero , GZ), which causes a second, faster pressure wave due to the "fairway" of the primary wave. At some distance from the hypocenter, the two unite to form a single, annularly propagating pressure wave which, compared to the pressure wave of a ground explosion, is weaker near the hypocenter, but considerably more destructive at a greater distance. According to Ernst Mach , this effect is also called the Mach effect or Mach reflection ( see illustration ). The military planning during the Cold War envisaged air explosions either to destroy large-scale unarmored targets such as industrial areas, warehouses, air bases or troop units, or to eliminate air targets such as aviation units or missiles.
The height of the explosion plays a crucial role when attacking extensive ground targets. The higher it takes place, the weaker the pressure wave that reaches the ground. At the same time, the floor area affected by the pressure wave increases. There is an optimal explosion height for every combination of a given explosion strength and overpressure of the pressure wave (or distance to the hypocenter). By choosing the optimal height, you can achieve greater damage in the largest possible area than with a soil explosion. The destroyed area can be up to twice as large. Another effect of an air explosion is the greater effect of the thermal radiation, since the angle of incidence is larger and the shielding by protruding buildings is reduced.
In the case of aerial targets, the reflected wave usually does not play a role, since the distance to the target is much smaller than the altitude. For this, the altitude dependence of air pressure and temperature must be taken into account more strongly. The use of atomic explosions to eliminate air targets is now largely obsolete and is being replaced by conventional weapons such as the MIM-104 Patriot missiles.
Air explosions contaminate the soil in the target area relatively little. In the case of soil explosions, there is a mixture with fission products or the generation of radionuclides in the soil material through neutron deposition , the fission products rise to great heights. The fallout is thus distributed over a large area, in the case of large explosions even globally. The total amount of radioactive residues, on the other hand, depends only slightly and the amount of fission products does not depend on the height of the explosion.
Soil explosion
The main features of a soil explosion are the radioactive contamination of large areas of land by fallout and the locally considerably stronger, but limited-range pressure wave. It is used to destroy bunker systems such as command centers, missile silos and dams. In particular, raised dams require the crater formation of the soil explosion. More information on the formation and size of the explosive craters depending on the explosive force can be found in the article Explosion craters .
Underground explosions

A distinction must be made between two cases of the underground explosion:
- Shallow explosions with massive crater formation and extremely strong fallout
- Explosions at great depths without releasing any fallout
As a possible use of nuclear explosions, the underground explosion has come back into the discussion lately. This type is particularly suitable for destroying underground command centers and bunker complexes. However, it is problematic to get the bomb intact deep enough underground. Unlike nuclear tests, where the bomb can be mined into the ground, the warhead would not penetrate deep enough to avoid radioactive fallout. Rather, it is sufficient to let the bomb penetrate a few meters into the ground, because this way the pressure wave reaches the bunker to be destroyed far better than with a surface explosion. One possibility to protect bunkers against this is therefore the movable storage of facilities and equipment within the bunker.
In nuclear tests , the deep underground explosion has been the common practice since above-ground nuclear tests were banned. The underground explosion at a sufficient depth has the advantage over the explosion at or above the surface that the radioactive products usually remain in the interior of the earth. However, underground nuclear tests occasionally resulted in "blowouts" through which radioactivity was released into the atmosphere. A long-term release of the fission products into the groundwater or into the sea cannot be ruled out based on current knowledge, especially in the case of test explosions in the porous rock of atolls , for example on the Pacific island of Mururoa .
Underwater explosions
The underwater explosion caused by nuclear weapons is used in particular to combat submarines or naval units. To this end, a wide variety of nuclear weapons have been built and tested by all the major nuclear powers. Are used torpedoes , depth charges or different types of missiles . Since pressure waves can propagate particularly well in water , underwater explosions as well as underground explosions can be detected almost worldwide with underwater microphones.
Due to the high speed of sound in water (around 1400 m / s), the pressure waves propagate more than four times as fast as in air. Due to the high density of the water and its low compressibility, the energy is transmitted to targets particularly effectively and over greater distances than in air. After the explosion, the gas bubble pulsates up to three times, expanding and contracting with decreasing intensity and frequency. The bladder becomes increasingly deformed as it ascends. When the hot gases reach the surface of the water, they and the water they carry with them create a considerable column of water and steam. The first pressure wave of a 100 kt explosion acts at a distance of 914 m (1000 yards ) with a pressure of more than 186 kPa . The pressure wave of an equally strong explosion in air would only generate a pressure of about 13.7 to 19.6 kPa over the same distance. The duration of the first pressure wave is only about two to three hundredths of a second under water compared to about a second in air. When the pressure wave reaches the surface of the water, it becomes visible there as a relatively minor disturbance. Due to the strongly differing physical properties of air and water, in particular due to the different wave resistances , the pressure wave can not propagate beyond the surface of the water, but the negative pressure component is reflected back downwards. The pressure wave is reflected to varying degrees on the sea floor, depending on its depth and nature. Due to all these peculiarities, a sea target is not only exposed to the main pressure wave, but also to the pressure waves caused by the pulsation of the gas bubble and the subsequent reflections of the pressure waves.
Similar to shallow underground explosions, large amounts of radioactive material are distributed in the immediate vicinity of the explosion site, although the direct nuclear radiation is largely absorbed. After some time, however, ocean currents lead to a worldwide distribution of the residues, while the local radiation rapidly decreases. More recent measurements at Bikini Atoll , where several underwater explosions were ignited, showed hardly any increased activity at the bottom of the lagoon.
High altitude explosion

ICBMs move over a large part of their trajectory in near-earth space . In order to destroy them, the USA and USSR planned the use of defense missiles with nuclear warheads, which should detonate in the immediate vicinity of the approaching warheads. Military satellites should be destroyed in the same way. At least the USA carried out several test explosions, some of which had unexpected effects on the upper atmosphere. The explosion of a small atomic bomb in the upper stratosphere more than 30 kilometers above the ground or in near-earth space has little effect on the pressure wave on the ground. Nevertheless, it can have serious effects on the civil and in some cases also on the military infrastructure, as a very strong electromagnetic pulse ( EMP ) is triggered. Above all, this can irreparably damage electronic devices with semiconductor components such as computers, televisions, radios or the electronic ignition in the car. In addition, even weak EMPs impair radio traffic. Therefore, the elimination of opposing electronic systems and disruption of communication are further possible objectives in addition to the direct combat against altitude targets.
Sequence of an explosion
The processes involved in the explosion of an atomic bomb range from the nuclear chain reaction to the formation of a fireball and pressure wave to the spread of the explosion cloud and the radioactive residues in the atmosphere. The time scales of the individual processes range from millionths of a second to several minutes. The explosion process can be roughly divided into
- Nuclear chain reaction (0 to 10 −6 seconds),
- Fireball and pressure wave formation (10 −6 to 0.1 seconds),
- Propagation of the pressure wave, cooling of the fireball (0.1 to 10 seconds),
- Formation of the mushroom cloud (seconds to minutes),
- Spread of the cloud, fallout (minutes to months).
The time scales are only approximate, as they depend heavily on the explosive force and the height of the explosion.
Nuclear energy release
The type and time scale of the energy release varies depending on the type of nuclear weapon . In the simplest case of a nuclear fission bomb with plutonium or highly enriched uranium , the chain reaction starts immediately after the critical mass is exceeded . Since the released neutrons at speeds of 1.4 · 10 7 meters per second cross the supercritical fissure mass, usually only about 10 to 20 centimeters in size, within 10 −8 seconds, and each time with a sufficiently high probability of causing another fission process, the average is The time between two generations of cleavage is also about 10 −8 seconds. Weapon-grade fissile materials must release an average of two or more neutrons per fission in order to ensure a sufficiently high growth rate. Since each split uranium or plutonium nucleus releases about 200 million electron volts (200 MeV, corresponds to about 32 picojoules), about 3 · 10 24 nuclear fission supplies an energy of 20 kilotons of TNT (corresponds to about 84 terajoules), the explosive power of the first atomic bomb. With a multiplication factor of 2 per generation - including the first neutron - so
Generations required. With an uninterrupted chain reaction, the release of energy is therefore complete after about 0.8 microseconds. Due to the exponential growth, most of the energy is released in the last generations. While the first 60 generations barely reach the energy of the conventional detonator charge of the bomb, after 77 generations the energy skyrockets to an equivalent of a thousand tons of TNT and the remaining 95% is released in the remaining five generations. After the nuclear fission process is complete, considerable energy is released through the decay of short-lived fission products.
In the case of the hydrogen bomb , this is followed by the phase of nuclear fusion , which takes a few microseconds, and, depending on the design, another nuclear fission, again induced by fast fusion neutrons, of a possible outer shell made of fissile material, which, however, causes large amounts of radioactive fallout . For this reason, this was also omitted from the Tsar bomb , although with a calculated 100 megatons of explosive force it would have been twice as powerful as the version actually used.
Fire bladder
Immediately after the nuclear fission is complete, the energy lies within the bomb shell in the form of
- electromagnetic waves ( photons ) as well
- kinetic energy of free electrons , neutrons and atomic nuclei (fission products)
in front.
Due to the enormous energy density , the temperatures inside the bomb rise rapidly to 60 to 100 million degrees Celsius. This corresponds to about 10,000 to 20,000 times the surface temperature of our sun (about 5500 degrees Celsius). The resulting “bubble” made up of hot fission products, the bomb jacket and the surrounding air is called a fire bubble . According to the Stefan-Boltzmann law, the radiation output per unit surface (also specific radiation, luminous intensity or radiation intensity ) is about a factor of 10 16 times greater than that of the sun. However, at this early stage, far from the radiation equilibrium, the fire bubble hardly behaves like a black body , so that this estimate is only very imprecise.
The first energy that leaves the bomb shell is gamma radiation , which hits the surrounding air at the speed of light and forms a thick fog of ozone and nitrogen oxides there . This fog means that the effective temperature determined from the measured radiation intensity is considerably below the true temperature at this early stage.
The fire bubble, also called isothermal sphere or by some sources also called “fireball”, expands suddenly and as soon as it leaves the bomb shell, it emits rays of light and heat into the environment. At this point in time it has a diameter of a few meters. At this stage, expansion takes place primarily through radiation, which is absorbed by air molecules and re-emitted and thus transferred to other air masses. The expansion of the hot gases, on the other hand, hardly plays a role.
The expanding fire bubble cools down to around 300,000 degrees Celsius within 100 microseconds. The thermal radiation output, which at this stage can at least roughly be estimated using the Stefan-Boltzmann law, has now reached its first maximum, especially since the ozone and nitrogen oxide mist itself has meanwhile largely been taken up by the fire bladder. At this point in time (in the case of a 20 kt explosion), a shock wave forms on the surface of the fire bubble , which is now around 25 meters in diameter , which initially spreads at around 30 kilometers per second and transfers part of its energy in the form of heat to the surrounding air. A second shock wave is created by the expansion of the bomb material; a little later it unites with the wave on the surface. How strong and how even this inner shock front is and when it unites with the outer one depends to a large extent on the mass and construction of the bomb.
As this internal shock front propagates through the fire bladder, the vaporized bomb material mixes with the ionized air. In the case of soil explosions, there is also evaporated soil, which means that the fire bladder is cooled down considerably compared to the air explosion. This effect is particularly strong when the height of the explosion is smaller than the radius of the external shock front at the moment of detachment; otherwise most of the evaporated soil will be blown aside.
Fireball and blast wave
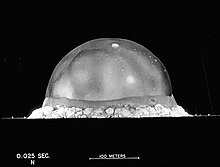
This compression heats the air to around 30,000 degrees Celsius (around five times the sun's surface temperature) - the actual fireball is formed, the luminous phenomenon of the explosion visible from the outside, which can be easily approximated in this phase by a black body. At this temperature, air is ionized and thus opaque, which weakens the luminosity of the considerably hotter and still expanding fire bladder or even shields it completely. With a 20 kT bomb, the luminosity reaches a temporary minimum after about 15 milliseconds. At this point the fireball has a diameter of about 180 meters.
As the fireball continues to expand, the impact front cools down further on its surface to around 3000 degrees Celsius and becomes transparent (“breakaway”). Behind it, the brightly shining fire bubble with a temperature of around 8000 degrees Celsius becomes visible again, which from now on is itself referred to as a fireball. The effective temperature increases, the fireball initially appears lighter again before the released fire bladder cools down until it goes out. This is how the double flash typical of atomic explosions occurs. At this point the fire bladder and fireball have almost reached their greatest dimensions. But the pressure wave spreads further. Unlike the zones with the same pressure level, the maximum expansion of the fireball does not scale with the cube root, but rather with it
The time to the second luminosity maximum t L or to the maximum size (before extinction and formation of the mushroom cloud ) t D also scales differently:
The fireball of a 20 kiloton explosion thus reaches a diameter of almost 500 meters after about one second, while the fireball of a 20 MT explosion grows to around 7 kilometers after 20 seconds.
The reason for the non-cubic scaling is that the radiation permeability for increasing optical thickness (larger fireball diameter) decreases exponentially instead of linearly and the thermal energy is therefore released somewhat more slowly than the pure cubic law. Above all, however, the collision front enveloping the hot fire bubble is optically denser in the case of stronger explosions and inhibits their radiation more strongly and for longer than in the case of lower explosive energies. The effective temperature of the fire ball surface in the second maximum determined from the radiation is therefore lower for larger explosions due to the conservation of energy . Taking into account the Stefan-Boltzmann law and the increasing proportion of thermal radiation with greater total energy (see section Effects of nuclear explosions ), the following approximately applies for the effective temperature and (relative) luminosity:
- ,
So about 8000 Kelvin at 20 kt, 7000 K at 1 Mt and 6000 K at 20 Mt of explosive force, while the luminosity is six times, 60 times and 300 times brighter than that of a 1-kt explosion. These relationships apply to air explosions at approximately sea level, but should only be treated as rough guidelines.
At higher altitudes, where the air density is lower, the final diameter is even larger than in denser air layers. However, due to the lower optical density, it radiates its energy faster. During high-altitude explosions, the air can be so thin that the much weaker shock front can barely cover the fire bladder. The thermal radiation is then released in a single pulse, the second maximum does not occur. At very high altitudes (over 80 kilometers) even the X-ray radiation leaving the warhead upwards can partially escape into space, while the part radiated downwards is absorbed below the explosion source and there forms a disk-shaped cloud of ionized gas. There is practically no pressure wave in such explosions.
For an ideal ground explosion in which the pressure wave is completely reflected on the surface, double the energy must be used in the shock wave-dominated phase of the fireball. In some cases, however, data from nuclear weapons tests show a reflectance of only approx. 70%, so that instead of double the 1.7-fold value should be used. This also applies to the strength of the pressure wave at a greater distance. With the same fireball volume, the same would apply to the late phase (when the shock front has become transparent). However, since the fire bladder is cooled to a greater extent in a ground explosion and accordingly expands less, the volume is smaller, so that the end radius may even be smaller than in the case of an air explosion. In particular, its luminosity is lower. This effect occurs more strongly with small explosive energies than with large ones.
Propagation of the pressure wave
After the impact-heated shell of the fireball has dissolved, the pressure wave (see also detonation wave ) continues to spread invisibly; in the process, it continues to compress the air and drive it away from the center of the explosion. The strength of the pressure wave decreases with distance: firstly due to the geometric thinning with increasing radius, secondly due to the conversion of the wave energy into heat and thirdly due to the increasing duration of the positive pressure phase as a result of the nonlinearity of shock waves . The dependence of the overpressure on the distance from a 1-kt explosion in an extensive, homogeneous air space is described by a standard curve. The mechanical energy share of around 50 percent of the total energy, which is typical for nuclear explosions, has already been taken into account in this. From the overpressure can over the Rankine-Hugoniot equations the speed of the pressure wave and the displaced air masses, and from the latter, the dynamic pressure (including back pressure ), should be calculated. The standard curve can be scaled for any explosive energy and atmospheric conditions. In this way, for any generation of blasts W, all lengths r are scaled with the cube root:
Example: In the case of an explosion with an explosive force equivalent to one megaton TNT (1000 kt), the base radius and detonation height must be scaled by a factor of 1000 1/3 = 10.
Explosions below the surface of the earth or water can also cause an air pressure wave. These are essentially generated by two main mechanisms. When the underground shock wave reaches the surface, some of the energy is transferred to the air. This pressure wave arrives close to the surface almost at the same time as the underground impulse that caused it. Due to the high density difference, however, this proportion is very small (see sound sources under water, which can hardly be heard on the surface). In the event of an explosion just below the surface, the fireball can also break through to the surface and trigger an air pressure wave due to the rapid expansion in the air. Their total strength can be estimated by a scaled explosive force W s , which decreases approximately exponentially with the depth d :
Where ρ is the density of the subsurface, and W 1/3 is the cube root scale for the depth d . W s can now be understood like an explosion directly on the surface. In a 1-kiloton underwater explosion (1 g / cm³), the atmospheric component of the pressure wave decreases by half about every nine meters. In the case of the well-known Baker test explosion at Bikini Atoll in 1946 ( W = 20 kt, d = 30 m under water), the pressure wave corresponded to a nuclear surface explosion of around 8 kt. The formula is only to be understood as a rough approximation.
In addition to the strength of the explosion, the ratio of the air pressure p to the mean air pressure at sea level p 0 = 101.325 kPa also depends on the factors
into the true pressure curve, which describes the dependence of the overpressure OP on the air pressure p and the distance to the explosion center R :
The temperature has no influence on the strength of the pressure wave, but it does influence the speed of propagation, which, like the classical speed of sound, scales with the square root of the temperature in Kelvin. If the target and the center of the explosion are at different heights, as is the case with air explosions, for example, the height of the target instead of the detonation height is decisive for scaling the overpressure .
The positive pressure phase is followed by a phase with negative pressure (negative pressure, "suction"). It arises due to the dilution of the gases behind the shock front, especially inside the fireball. The duration of this phase is generally longer than that of the positive, but the amount of negative pressure is less than that of the positive pressure maximum.
The negative pressure phase is also responsible for the typical condensation rings that spread around the source of the explosion when the air humidity is high and above all determined the typical appearance of the test explosions in the Pacific, also known as a Wilson cloud . The drop in pressure leads - despite the enormous heat radiation of the fireball - to the cooling of the air and thus to condensation of the moisture. The mist disappears as soon as the pressure returns to normal. Similar phenomena can also be observed in conventional explosions or in supersonic aircraft ( cloud disc effect ).
Mushroom cloud (mushroom cloud)


After the pressure wave “breaks away”, the fireball continues to cool down and begins to rise due to convection . It pulls up dust and ashes with it. The well-known mushroom cloud ("atomic mushroom") is created.
The maximum height of the mushroom cloud depends mainly on the explosion energy, also on the detonation height and the weather conditions. The peak height of the explosion cloud of a near-surface explosion in the kt range is only a few kilometers, while the cloud of the 57 Mt strong " Tsar bomb ", the strongest bomb ever detonated, rose 64 kilometers high. For low explosive energies (below about 10 kt) the final height and width of the cloud scale with the cube root of the energy, while for larger explosions the stratification of the earth's atmosphere has a significant influence on the cloud size and shape. In particular in the stratosphere, the prevailing temperature inversion inhibits the ascent of the cloud. In the case of very high explosion energies, on the other hand, the large volume of the cloud, which expands even further with decreasing pressure at great heights, leads to a sharp increase in height (see figure).
A few minutes after the explosion, the head of the mushroom cloud stabilizes at a certain height; in the case of large explosions (over about 1 Mt), a greater height can be reached for a short time. After reaching the final height, the cloud can only spread to the side; therefore the width increases very sharply with large explosive forces. According to simulations, an explosion of more than about 1000 megatons would no longer stabilize, but would expand into space as a plume . Atmospheric explosions of this size have so far only been observed during the impact of Comet Shoemaker-Levy 9 on Jupiter .
In some atomic bomb explosions, the mushroom cloud shows a glowing ring-shaped tube. This arises from the fact that the rising fireball gets into a toroidal rotation due to the friction with the surrounding air, similar to the formation of smoke rings , and the hot (and thus brightly shining) gases collect in this ring.
In many nuclear tests you can see several parallel smoke streaks next to the detonation mushroom. These are not an effect of the nuclear explosion, but come from previously launched smoke track missiles, the traces of which are used to measure the pressure wave.
Effects of nuclear explosions

An atomic bomb explosion has the following effects on its surroundings:
- Pressure wave, which is similar to normal explosions, but considerably stronger (proportion of total energy 40-60%)
- direct thermal radiation ( UV and infrared radiation as well as visible light, proportion of the total energy 30–50%)
- direct ionizing radiation (especially neutron, gamma and x-ray radiation, share of total energy about 5%)
- indirect radioactivity from fallout particles (depending on the type of bomb)
- Nuclear electromagnetic pulse (NEMP; consequence of direct ionizing radiation)
The proportions of the individual effects in the total energy vary with the explosive power and the mass and construction of the bomb. In general, the proportion of thermal radiation increases with greater explosive power or lower bomb weight, while the proportion of pressure waves decreases. The direct ionizing radiation - this includes neutrons from the fission processes and gamma rays from the decay of very short-lived fission products, but also X-rays, which arise as a result of the high temperatures in the first luminous maximum - is released above all during detonations in the air and at high altitudes, underwater and Underground explosions, however, inhibited. Furthermore, the proportion of indirect nuclear radiation is almost exclusively caused by the fission products and is therefore greatest in pure nuclear fission bombs (around 10 percent of the total energy). An exception is the theoretical cobalt bomb , of which not a single example is known. In general, however, indirect radiation is not counted as explosive energy, as it is released long after the actual explosion.
Consequences of the pressure wave
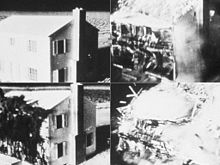
The greatest damage is caused in built-up regions (cities) by the blast wave. As described above, it causes sudden strong pressure fluctuations (static overpressure and underpressure) and hurricane-like winds (dynamic pressure). The static overpressure mainly destroys closed buildings with large cavities, i.e. especially houses, while the hurricane-like wind "blows" people, animals, trees and light structures. The static negative pressure that follows the overpressure phase and is associated with weaker winds in the direction of the explosion center is usually negligible in terms of damage. Above all, the maximum pressure plays a role: If the load limit z. B. exceeded for the concrete walls of a building, the break occurs within a very short time. Nevertheless, the duration of the pressure wave also has a certain significance. According to A. Bühl (1972), an overpressure of 0.3 atü (approx. 30 kPa) of an explosion in the megaton range has an effect comparable to that of a pressure wave of 0.5 atü (approx. 50 kPa) of an explosion in the kiloton range. This effect is difficult to calculate as it is essentially related to the construction, size, shape and spatial orientation of the house and is therefore neglected in the following.
The following table gives an impression of the effects of the pressure wave. However, it should be noted that the effect on people includes all effects of the explosion, including thermal and radiological effects. Regarding the units of pressure used, it should be noted that a large part of the sources on the subject come from the USA, where the unit pound-force per square inch (psi) is very common, and many information is therefore in psi instead of the SI -compliant kilopascals (kPa). In the following, both units are used side by side.
Pressure amplitude | Typical effects on urban areas | |
---|---|---|
psi | kPa | |
0.2 | 1.4 | Typical window panes break |
1 | 6.9 | Windows smashed, injuries from splinters possible |
3 | 21st | Residential houses (lightweight construction) badly damaged or destroyed, numerous seriously injured, isolated fatalities |
5 | 35 | Destruction of most of the unreinforced buildings, numerous deaths |
10 | 69 | Destruction or serious damage to reinforced concrete structures, death of most of the residents |
20th | 138 | Destruction or serious damage even to heavy concrete structures, hardly any survivors (hypocenter of Hiroshima : approx. 30 psi) |
50 | 350 | Complete destruction of all structures above ground (Hypocenter of Nagasaki : about 60 psi) |
300 | 2000 | Complete leveling of the landscape (hypocenter of the " Tsar bomb ") |
The relationship between the maximized distance from the hypocenter GR OP ("ground range"), within which the given overpressure OP occurs, and the optimal detonation height H OP is approximately given for an explosion of one kiloton TNT equivalent
By this choice of H OP maximized radius GR OP can be estimated by the following approximation:
For other explosive energies, the mentioned cube root rule applies. These formulas are mathematically accurate to ± 20 percent for H and ± 10 percent for GR in the range from 0.1 to 10,000 psi , but neglecting the atmospheric pressure variation and for flat terrain. This simplification is still plausible for detonation heights below about 6000 meters (this corresponds to about half the air pressure on the ground).
The militarily interesting range for air detonations is between 5 psi and about 50 psi (35 kPa to 350 kPa). Typical utility buildings such as factories, barracks or other not particularly reinforced buildings are destroyed at an overpressure of about 5 psi or the corresponding dynamic pressure; therefore, the greatest destruction is to be expected in urban areas for H 5 psi . However, heavy concrete structures or armored vehicles can withstand far greater pressures. Above a targeted pressure of 50 psi, however, the reinforcement effect is negligible and ground detonation is preferable. The atomic bombs dropped on Hiroshima and Nagasaki in World War II had explosive energies of 15 kt ( Little Boy ) and 21 kt ( Fat Man ) and detonated at heights of 580 and 503 meters, which, according to the above formulas, give a maximized radius of 10 psi and 19 psi (68 kPa or 132 kPa). The reason for this conservative choice (“optimal” for 5 psi would be around 800 meters or 900 meters) was the uncertainty of the predicted explosive force; In addition, certain strategically important structures such as bridges can withstand higher pressures.
Most deaths outside of buildings are due to dynamic pressure. People and animals are thrown through the air, loose objects can have the effect of projectiles. Incidentally, this is also the greatest danger in strong cyclones such as tornadoes . The blast wave is also responsible for fires caused by the destruction of gas pipes, power cables and fuel systems.
In the case of a ground detonation, the enormous pressure also causes the formation of an explosion crater. Most of the soil from the crater is deposited on the crater rim; However, soil in the immediate vicinity of the explosive device is pulverized and enriched with radioactive residues from the fissile material. This contributes significantly to the fallout.
Consequences of light and heat radiation
About a third of the energy released by a nuclear explosion is converted into thermal radiation (including light). Since thermal radiation propagates through the atmosphere at the speed of light, flashes of light and thermal radiation occur a few seconds before the pressure wave arrives. If you look in the direction of the explosion immediately during or shortly after the detonation, the enormous luminance can lead to temporary or permanent blindness even at great distances, as the light of the fireball is focused on the retina from the lens of the eye and only at a greater distance the focal spot becomes smaller, but, apart from absorption in the air, the irradiance in the focal spot on the retina hardly decreases.
The emitted thermal radiation causes burns to the skin, which decrease with greater distance from the ground zero point. In the hypocenter, the heat development is generally so strong that almost all matter evaporates. The distances at which burns occur are very different, since high humidity or dust particles weaken the heat radiation, while snow, ice or light sand as well as a cloud cover above the explosion point can increase it locally by more than twice. With a clear sky and average visibility (20 kilometers), an air explosion of 1 Mt causes third-degree burns within a radius of up to 12 kilometers, second-degree up to 15 kilometers and first-degree up to 19 kilometers. There is no chance of survival within the actual explosion radius. For example, inexplicable white spots were initially found in Hiroshima and Nagasaki after the explosions. These were the shadows of people whose bodies protected the ground from the scorching before they were thrown away by the shock wave.
In addition, all flammable substances are ignited in a wide area. The resulting fires occur before the pressure wave arrives and are partially extinguished by it, but can also be fanned into enormous firestorms by the dynamically occurring winds.
Consequences of direct nuclear radiation
All nuclear weapons emit ionizing radiation during the explosion. The ionizing radiation that is released during the first minute after ignition is called direct or initial radiation. It essentially consists of three components that have a relatively long range in the air:
- Neutron radiation from the nuclear fission and nuclear fusion processes,
- Gamma radiation from the nuclear processes and the excitation of nuclei in the air,
- Gamma radiation from the decay processes of short-lived fission products.
Furthermore, beta and alpha rays mainly from decay processes should be mentioned, which, due to their short range in air, mainly contribute to indirect radiation (mainly via the contamination of breath, water and food by the fallout), but hardly contribute to direct nuclear radiation. The radiation dose D increases due to the absorption in air ( exponential ) and the geometric distribution (quadratic) approximately according to the relationship
with the distance r from the center of the explosion and only has a relevant effect with smaller explosive forces up to about 50 kilotons, since with larger explosive forces the thermal radiation (absorbed to a much lesser extent by the air) and the pressure wave are already fatal. In the explosions in Hiroshima and Nagasaki, for example, direct nuclear radiation claimed the highest number of deaths, measured against its share of just a few percent of the total energy. Those affected, who took an equivalent dose of around 1 Sv ( Sievert ), fell ill with so-called radiation sickness . From a dose of 6 Sv people have little chance of survival, at 10 Sv death occurs within one to two weeks.
The direct nuclear radiation is only effective during the atomic explosion for a period of about one minute - albeit very strongly, with most of the radiation being released within the first fractions of a second. If an affected person can partially or completely shield the direct nuclear radiation with suitable protection, his risk of radiation sickness is considerably reduced. In Hiroshima, for example, people who were protected by a concrete wall at the moment of the explosion survived, while unprotected people died of radiation sickness only a few meters away from the obstacle.
Consequences of the fallout
As a fallout , a mixture of various radioactive substances and dust is known that fails or the passage of time from the mushroom cloud is washed out by rain. Most fallout is generated during detonations at or near the ground, where radioactively contaminated dust is whirled up by the pressure wave and transported into the atmosphere along with the mushroom cloud. In contrast to the fine residues of the bomb, which are even distributed worldwide over several months, the coarser dust particles largely fall out after a few hours or even minutes. In the case of air explosions, this short-term component is largely or completely absent.
The precipitation of the substances takes place over a very large area depending on the prevailing wind direction and wind speed. The greatest amount of contaminated particles fall to the ground around the hypocenter, especially in the event of a ground explosion, and the degree of contamination decreases with increasing distance. Nevertheless, higher concentrations, so-called hotspots , can occur locally , for example through rainfalls enriched with contaminated dust.
If the fallout is visible as a thin layer of dust, the radiation is often strong enough to cause immediate damage to health. If a certain dose is reached, the affected person will suffer severe radiation damage , which will either result in radiation sickness or even death.
Consequences of the electromagnetic pulse
The electromagnetic pulse (EMP), in particular NEMP (nuclear electromagnetic pulse), is a short-term, very strong electromagnetic field that occurs when X-rays or gamma rays interact with electrons in air molecules ( Compton effect ). Since the electrons have a much smaller mass than the atomic nucleus, they are influenced to a much greater extent by the Compton effect and are driven away radially from the explosion site. This leads to a slightly asymmetrical electrical charge separation due to the atmospheric density gradient and thus to an electrical dipole moment . The acceleration of the electrons also causes magnetic fields , so that electromagnetic waves are generated. The EMP differs from ordinary radio waves in two ways:
- Due to its high amplitude , the EMP is able to induce voltages in the kilovolt range in metal structures of great spatial expansion .
- The energy is released as a single pulse with a duration in the microsecond range and a rise time in the order of one nanosecond.
The EMP is thus similar to a lightning strike in terms of the effects on electrical lines, but the voltage rise is significantly steeper than with natural lightning. (That is why lightning protection systems do not respond due to their inertia.)
All electrical or electronic devices and systems with long cables or antennas and sensitive components such as semiconductors and capacitors are damaged by the EMP. These include the power supply (overhead line network), telephone networks, household appliances, radio and television stations. Only radios with very short antennas are less affected.
Depending on the type of ignition, a distinction is made between an Endo-NEMP, which is created by an explosion within the atmosphere between about 30 and 100 kilometers altitude, and the Exo-NEMP, in which the explosive device explodes in space. The variants differ greatly in terms of their strength and size. With the Endo-NEMP, for example, the gamma and particle beams are still absorbed in the vicinity of the explosion site, while with Exo-NEMP the particle density at the detonation height is so low that the beams can travel hundreds or even thousands of kilometers before they are absorbed by air molecules become. In addition, the geometric distance to the earth's horizon is greater at high altitude . As a result, an entire continent can be affected by the effects, although the pulse is much weaker than with the locally concentrated Endo-NEMP.
Main impact table
The most important effects of nuclear explosions are summarized here in tabular form. The information comes from the sources listed below. The table applies to typical air explosions under the following conditions:
- Flat terrain,
- Visibility : 20 kilometers,
- Explosion Height: Optimized for 15 psi (about 103 kPa),
- “Normal” explosives, especially no neutron bombs or other types with particularly strong or weak radiation.
The hypocenter overpressure is approximately 42 psi (290 kPa) in all cases.
Effect up to GR / km | Explosive energy / explosion height | ||||
---|---|---|---|---|---|
1 kt / 200 m | 20 kt / 540 m | 1 mt / 2.0 km | 20 mt / 5.4 km | ||
Pressure effect | |||||
Total destruction (20 psi ≈ 140 kPa) | 0.2 | 0.6 | 2.4 | 6.4 | |
Extensive destruction (5 psi ≈ 35 kPa) | 0.6 | 1.7 | 6.2 | 17th | |
Moderate civil damage (1 psi ≈ 7 kPa) | 1.7 | 4.7 | 17th | 47 | |
Thermal effect | |||||
Strong fire effect | 0.5 | 2.0 | 10 | 30th | |
3rd degree burns | 0.6 | 2.5 | 12 | 38 | |
2nd degree burns | 0.8 | 3.2 | 15th | 44 | |
1st degree burns | 1.1 | 4.2 | 19th | 53 | |
Effect of ionizing direct radiation (room diagonal 1 SR / km) | |||||
Deadly 2 total dose (neutrons and γ-rays) | 0.8 | 1.4 | 2.3 | 4.7 | |
Acutely harmful 2 total dose | 1.2 | 1.8 | 2.9 | 5.4 |
Consequences of a nuclear war
The consequences of an intercontinental nuclear war cannot be understood by simply adding together numerous atom bomb explosions. Rather, due to the large area coverage, further effects are to be expected:
- Breakdown of national or continental populations and society.
- Long-term increased occurrence of malformations and cancer in all living things due to the mutagenic effects of ionizing radiation and the longevity of some radioactive nuclides
- Destruction or severe damage to entire ecosystems , mass extinction
- Climate changes due to reduced solar radiation from smoke and dust ( nuclear winter )
- Complete or extensive destruction of the entire infrastructure of the countries involved, made recovery more difficult due to the loss of facilities for production and raw material extraction
There is disagreement about the extent of the individual consequences, because a reliable prediction is hardly possible due to the complexity of the global climate alone and especially of biological and social systems. Therefore, this information should be viewed very generally and with critical distance.
Legal situation in Germany
According to Section 307 of the Criminal Code, causing an explosion by nuclear energy is punishable if there is a threat to other life or other things.
Section 307 Causing an Explosion Using Nuclear Energy
- Anyone who undertakes to cause an explosion by releasing nuclear energy and thereby endangering the life or limb of another person or property of significant value will be punished with imprisonment for no less than five years.
- Anyone who causes an explosion by releasing nuclear energy and thereby negligently endangering the life or limb of another person or property of significant value will be punished with imprisonment from one year to ten years.
- If the perpetrator causes the death of another person, at least carelessly, then the punishment is
- in the cases of paragraph 1 life imprisonment or imprisonment not less than ten years,
- in the cases of paragraph 2 imprisonment not less than five years.
- Anyone who acts negligently in the cases of paragraph 2 and negligently causes the risk is punished with imprisonment for up to three years or with a fine.
According to Section 17 (1) of the War Weapons Control Act (KrWaffKontrG) it is forbidden to “develop, manufacture, trade with them, acquire them from another person or leave them to someone else, import them, export them, carry them through the federal territory or otherwise to move the federal territory or out of the federal territory or to otherwise exercise actual power over them ". The offense against it is punished with a prison sentence of one to five years. (Section 19 (1) KrWaffKontrG)
See also
literature
- Nuclear explosions and their effects . Introduction by Carl Friedrich von Weizsäcker , Fischer-Bücherei Nr. 386, 1961.
- Samuel Glasstone, Philip J. Dolan: The Effects of Nuclear Weapons (third edition) , United States Government Printing Office , 1977. One of the most extensive publications on this subject (available in antiquarian or PDF format ).
- George C. Messenger, Milton S. Ash: The effects of radiation on electronic systems . Van Nostrand Reinhold, New York 1986, ISBN 0-442-25417-2 .
- Joseph Rotblat : Radiation Effects when Using Nuclear Weapons , Berlin 1996, ISBN 3-87061-544-3
- Operation Ivy, Operation Castle, papers on the evaluation of nuclear tests in the Marshall Islands
- William C. Bell, Cham E. Dallas: Vulnerability of populations and the urban health care system to nuclear attack - examples from four American cities (PDF), International Journal of Health Geographics 2007, 6: 5, ISSN 1476-072X
- Alfons Bühl, Atomwaffen , Osang Verlag, 3rd edition, Bad Honnef 1972
- Michael Light: 100 Sonnen , Munich: Knesebeck 2003 ISBN 3-89660-190-3 , illustrated book
Web links
- Nuclear Weapon Archive - Information on nuclear weapons, nuclear tests and nuclear policy
- Software for calculating explosion effects - alongside Glasstone and Dolan (1977) the basis of the data and formulas
- cddc.vt.edu: Trinity Atomic Web Site (archive version )
- Nuclear Explosions Database
- "1945–1998" - video that shows all nuclear weapons explosions from 1945 to 1998 on a stylized world map.
Individual evidence
- ↑ Miller / Jordan: Modern Submarines. Verlag Stocker Schmid / Motorbuchverlag, 4th edition, 1999, ISBN 3-7276-7088-6 , pp. 89-92.