Tests of general relativity
Tests of general relativity are carried out to test Albert Einstein's standard model for describing gravity , Albert Einstein's general theory of relativity (ART) .
At the time of its introduction in 1915, the ART had no empirical basis. Rather, it was originally very satisfactory for philosophical reasons, since it fulfilled the principle of equivalence and contained Newton's law of gravitation and the special theory of relativity as borderline cases. Experimentally, all that was known was that it could explain the “abnormal” perihelion of Mercury . It was proven as early as 1919 that light in the gravitational field is deflected according to the ART. However, it took until 1959 before it was possible to test the predictions of the ART in the area of weak gravitational fields, whereby possible deviations from the theory could be precisely determined. It was not until 1974 that studying binary pulsars made it possible to research much stronger gravitational fields than exist in the solar system . Finally, the study of strong gravitational fields also took place in connection with black holes and quasars . Naturally, observations are very difficult here, but the results have so far been consistent with the predictions of the ART.
According to the equivalence principle, the Lorentz invariance valid in the special theory of relativity must be fulfilled locally (“Local Lorentz invariance”). For the corresponding experiments see Tests of the special theory of relativity .
Classic tests
In 1916 Einstein proposed three ART tests, which were later referred to as "the classic ART tests":
- the perihelion of Mercury's orbit
- the deflection of light in the sun's gravitational field
- the gravitational redshift of light.
Perihelion of Mercury
In Newtonian physics , objects in a two-body system , i.e. two celestial bodies orbiting each other, describe an ellipse with the center of gravity as their focal point . The point of closest approach, the perihelion , is inherently immobile. In the solar system, however, a number of effects cause the perihelion of the planets to rotate around the sun. The main cause of this is the presence of other planets perturbing their orbits with one another. Another, much smaller effect is the flattening of the sun. Originally, the measurements of the planetary orbits were carried out by conventional telescopes , but today much more precise measurements are made with radar .
According to Newton's theory of gravity, a perihelion rotation of about 531 "( arcseconds ) per century would be expected. In 1859, Urbain Le Verrier recognized that the perihelion rotation of Mercury was different from that resulting from the Newtonian effects. His analysis of the transits of Mercury over the Sun disk from 1697 to 1848 showed a deviation from Newton's theory of about 38 "per tropical century (later changed to 43"). A number of ad hoc hypotheses and ultimately wrong solutions have been proposed. In GTR, however, the remaining one is used Rotation, or the change in the orientation of the orbital ellipse in its orbital plane, caused by the curvature of space . Einstein was able to show that the GTR is very close in agreement with the observed amount of about 43 "of the perihelion displacement, which is of considerable weight for the Acceptance of the ART was.
The other planets are also subject to perihelion rotations, but they have lower orbital velocities and fewer eccentric orbits, so their displacements are smaller and harder to find. For example, the perihelion rotation of Earth's orbit due to the ART is about 5 "per century. The perihelion shifts of binary pulsar systems were also measured, being about 4.2 ° per year at PSR 1913 + 16, for example. These observations are consistent with the ART.
Deflection of light by the sun
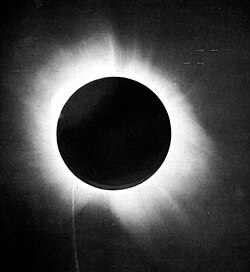
Henry Cavendish (1784 in an unpublished manuscript) and Johann Georg von Soldner (1801) pointed out that Newton's theory of gravity predicts a deflection of starlight around celestial bodies, provided that light is understood as a particle with mass . Almost the same value as Soldner was derived from Einstein based on the assumption of the principle of equivalence alone. In 1915, however, taking into account the space-time curvature in the GTR, he noticed that this was only half of the correct value.
The first observation of light deflection was made when observing the change in position of stars when they are very close to the sun on the celestial sphere . The observations were made by Arthur Stanley Eddington and Frank Dyson during the total solar eclipse of May 29, 1919 . The result was seen as spectacular news and appeared on the front pages of many major newspapers. It made Einstein and his theory world famous. When asked about his reaction if the ART had not been confirmed by Eddington, Einstein said jokingly: "I would have been sorry for God - the theory is correct".
While the original accuracy was not particularly high, a modern re-analysis of the data showed that Eddington's analysis was essentially correct. The measurement was repeated by a team from Lick Observatory in 1922, and also in 1973 by a team from the University of Texas at Austin , with results consistent with those of 1919. A really great precision, however, only showed the measurements with the help of radio astronomy , beginning in the 1960s, which were able to dispel the last doubts about the validity of the ART values.
In addition to the deflection by the sun, Einstein rings are also an example of the deflection of light.
Gravitational redshift of light
Einstein predicted the gravitational redshift of light as a consequence of the equivalence principle as early as 1907, but the measurement of this redshift proved to be very difficult. Although it was approximately measured by Walter Sydney Adams as early as 1925, a clear measurement was only made by the Pound-Rebka experiment (1959). There, the relative redshift of two sources at the top and bottom of the Jefferson Tower at Harvard University was measured using the Mössbauer effect . The result was in excellent agreement with the ART and one of the first precision measurements of its predictions.
Modern tests
The modern era of tests of general relativity was further fueled by Robert Henry Dicke and Leonard Schiff , who developed a scheme for testing GTR. They emphasized the importance not only of the classic tests, but also of zero results, i.e. H. the search for effects that can in principle occur in a gravitation theory, but not in the GTR. Other important theoretical developments concern the consideration of alternatives to the GTR, especially scalar tensor theories such as the Brans-Dicke theory or the parametrized post-Newtonian formalism, a test theory with which deviations from the GTR as well as effects related to the Principle of equivalence can be quantified.
Post-Newtonian gravity tests
Due to the lack of useful alternative theories, the scope for testing GTR was originally limited because it was not clear what kind of tests differentiated it from other theories. At that time, the GTR was the only relativistic theory of gravity that agreed with the special theory of relativity (in its area of validity) and the observations. That changed with the introduction of the Brans-Dicke theory in 1960. In a certain sense, this theory can be described as simpler, since it contains no dimensional constants and is compatible with Mach's principle and Paul Dirac's Large Number Hypothesis , i.e. with two philosophical ideas who have proven influential in the history of GTR. This eventually led to the development of the parametrized post-Newtonian formalism (PPN) by Kenneth Nordtvedt and Clifford Will , which contains all possible deviations from Newton's law of gravity for first order quantities to v / c (where v is the speed of the object and c is the speed of light) . This approximation makes it possible to systematically analyze the possible deviations from the ART for slowly moving objects in weak gravitational fields. Great experimental efforts have been made to limit the post-Newtonian parameters - with the result that deviations from the GTR are only possible within very narrow limits.
The experiments to check the gravitational lens effects and light delays limit the same post-Newtonian parameter, the so-called Eddington parameter γ , which is a direct parameterization of the size of the light deflection by a gravitational source. It is equal to 1 for the GTR, and takes different values in other theories. It is the best determined of the ten post-Newtonian parameters, but tests also exist for narrowing down the other parameters. These include, for example, the perihelion of Mercury and the tests of the strong equivalence principle.
Gravitational lensing effect
The gravitational lensing effect has been observed at distant astrophysical sources, but the conditions are difficult to control experimentally and it is uncertain how the results will be classified in the context of the GTR. The most precise tests correspond to Eddington's measurements from 1919: They measure the deflection of radiation from a distant source by the sun. The sources that can be most accurately analyzed are distant radio sources, especially quasars . The directional accuracy of all telescopes is basically limited by diffraction , and for radio telescopes this is also the practical limit. An important improvement in obtaining position data of high accuracy (in the range of milliseconds to micro-arcseconds) has been achieved through the combination of radio telescopes around the world ( VLBI ). With this technology, the phase information of the radio signals measured with telescopes is linked over long distances through radio observations. In 2009, such telescopes measured the deflections of radio waves by the sun with extremely high accuracy, with the amount of the deflection resulting from the ART measured to an accuracy of 0.03 percent. At this level, systematic effects must be carefully considered in order to determine the exact position of the earth telescopes. Some important effects are nutation , rotation , atmospheric refraction , tectonic displacement, and tidal waves. Another effect is the refraction of radio waves by the solar corona . It helps that this effect has a characteristic spectrum , while gravitational deflections are independent of the wavelength . Consequently, careful analysis of measurements at different frequencies can reduce this source of error.
The entire sky is slightly distorted by the gravitational deflection of light (caused by the sun) (except in the opposite direction of the sun). This effect was observed by Hipparcos , an astrometric satellite of the European Space Agency . He measured the position of about 100,000 stars. During the entire mission, 3.5 million relative positions were determined, each with an average accuracy of 3 milli-arcseconds (the accuracy for a star of size 8 to 9). Since the gravitational deflection perpendicular to the earth-sun direction is already 4.07 milli-arcseconds, corrections are necessary for practically all stars. Without systematic effects, the 3 milli-arcsecond error of a single observation can be reduced to the square roots of the number of positions, resulting in a precision of 0.0016 milli-arcseconds. Systematic effects limit the accuracy of determining the size of the overall effect to 0.3 percent.
Tests for delaying the time of flight
Irwin I. Shapiro suggested a test that can be performed within the solar system and is sometimes referred to as the fourth "classic" test of GTR. He calculated a relativistic time delay ( Shapiro delay ) for the two-way time (round trip) of radar signals that are reflected from other planets. The mere curvature of the path of a photon passing close to the sun is too small to produce an observable delay effect (when the two-way time is compared to the time it takes for a photon to move in a straight line), however, the ART says a delay which increases continuously due to the gravitational time dilation as the photon passes close to the sun. The observation of radar reflections from Mercury and Venus , immediately before and after they were eclipsed by the sun, shows an agreement with the ART with a maximum deviation of 5%. More recently, a similar experiment was carried out with the Cassini satellite , the maximum deviation from the ART of which was only 0.002%. With the VLBI , speed-dependent ( gravitomagnetic ) corrections of the Shapiro delay in the field of the moving Jupiter and Saturn were measured.
The equivalence principle
The equivalence principle says in its simplest form that the trajectories of a falling body in a gravitational field should be independent of its mass and internal structure, provided they are small enough that they are not influenced by the environment or by tidal forces . This principle was confirmed with great precision by the Eötvös experiment with a torsion balance , where different accelerations of different masses were searched for. Limits regarding this effect and the existence of a composition-dependent fifth force or a gravitational Yukawa interaction are already very narrow.
The strong equivalence principle states, among other things, that falling bodies that are held together by their gravitational forces, such as stars, planets or black holes , are subject to the same trajectories in a gravitational field, provided that the same conditions are met. This is known as the Nordtvedt Effect , and has been most accurately confirmed by Lunar Laser Ranging . Since 1969, it has been used to measure the distance of some stations on earth with respect to the moon, with centimeter precision being achieved. Thereby a strong limitation of various post-Newtonian parameters was achieved.
Another part of the strong equivalence principle is the condition that the Newtonian gravitational constant is invariable in time and has the same value everywhere in the universe. There are many independent measurements that will limit how much it can vary, but one of the best is based on Lunar Laser Ranging. These measurements showed that the gravitational constant - should it change against the current view - cannot change by more than 10 −11 per year.
Gravitational redshift
The first of the classical tests discussed above, gravitational redshift, is a simple consequence of the principle of equivalence and was predicted by Einstein in 1907. In and of itself, it is not a test in the form of the post-Newtonian tests, since any theory that implies the principle of equivalence must also predict this effect. Nevertheless, the proof of this effect is an important support for the relativistic conception of gravity, since the lack of gravitational redshift would have clearly contradicted the theory of relativity. The first observation of this effect was the measurement of the spectral shift of the white dwarf star Sirius B by Adams (1925). Although these and later measurements of the spectral shift of other white dwarf stars agreed with the predictions of the GTR, it could be argued that the shift may have other causes, which is why experimental confirmation using terrestrial sources would be preferable.
The experimental confirmation of the gravitational redshift using terrestrial sources took several decades, since the effect here is much smaller and therefore very precise frequency measurements are necessary. It was first demonstrated experimentally in 1960 using the Mössbauer effect . This allows photons of gamma radiation to be generated with a very narrow line width . The experiment performed by Robert Pound and Glen Rebka , later improved by Pound and Snyder, is called the Pound-Rebka experiment . The frequency shift could be measured with an accuracy of 1%. The blue shift of the falling photons can be calculated by assuming that they have an equivalent mass according to their frequency E = hf (where h is Planck's constant ) and E = mc² - a result of special relativity. Such simple deductions, however, ignore the fact that in the GTR clock rate rates are compared rather than energies. In other words, the “higher energy” of the photon after it has fallen can also be attributed to the slower rate of clocks in lower regions of the gravitational potential . To fully confirm the ART, it is important to show that the rate of arrival of the photons is greater than the rate of emission. A very accurate redshift experiment was conducted in 1976, taking a hydrogen maser clock in a rocket to an altitude of 10,000 km and comparing its rate with an identical clock on the surface of the earth. The gravitational redshift was measured with an accuracy of 0.007%.
Although GPS was not designed as a test of fundamental physics, gravitational redshift must be taken into account in the operation of this navigation system, with physicists analyzing the time data to verify statements made by various theories. When the first satellite was launched, some engineers ignored the prediction of a noticeable gravitational time dilation, so the satellite was launched without an appropriate clock setting. The clocks showed the expected shift according to the theory of relativity of 38 microseconds per day. The deviation rate is sufficient to substantially impair the functions of the GPS within hours if it is not taken into account.
Other precision tests in this context have been carried out, for example, with the satellite Gravity Probe A (1976), which has shown that gravitational and velocity effects influence the ability to synchronize the rate of clocks in orbit. Atomic clocks were used in airplanes in the Hafele-Keating experiment (1971) and in the Maryland experiment , which also confirmed the relativistic effects of gravity and speed.
By using optical clocks , the accuracy has been increased to such an extent that the gravitational time dilation could be measured even at distances of less than one meter. Chou et al. (2010) use Al + ions as clocks. While one ion was at rest, the other was raised 33 cm. The redshift determined corresponded to a height difference of 37 ± 15 cm, which is in very good agreement with the theory.
Lense thirring effect
According to the Lense-Thirring effect , small precessions of the orbit of a test particle should result, which moves around a central, rotating mass, for example a planet or a star. Corresponding tests were carried out with the LAGEOS satellites, however some aspects of these experiments remained controversial. The effect could also have been measured with the Mars Global Surveyor , but this was not without controversy.
The Gravity Probe B satellite, which was launched in 2004 and was in function until 2005, was finally able to demonstrate this effect for the first time without a doubt. In this experiment four quartz balls coated with a superconductor and about the size of table tennis balls were used. The data analyzes continued until 2011 due to the high level of interference and difficulties in correctly modeling the interference. Only then could a meaningful signal be found. Researchers at Stanford University announced on May 4, 2011 that they measured the Lense-Thirring effect relative to the distant star IM Pegasi . The geodetic effect could be proven to an accuracy of 0.2 percent (measured value: −6601.8 ± 18.3 milli-arcseconds / year, ART value: −6606.1 mas / year) and the lens-thirring effect was up to Measured to 37 milli-arcseconds with an error margin of 19 percent (measured value: -37.2 ± 7.2 mas / year, ART value: -39.2 mas / year). For comparison: a milli-arcsecond corresponds to the width of a human hair from a distance of 16 km.
In addition, experiments are carried out to demonstrate the Lense-Thirring effect of the sun on the perihelion rotations of the inner planets. Another consequence of the effect would be that the orbital plane of the stars orbiting near a supermassive black hole would be made to precession around the axis of rotation of the black hole. This effect should become verifiable in the next few years through astrometric observation of the stars in the center of the Milky Way. By comparing the rate of orbital precession of two stars in different orbits, it should also be possible in principle to confirm the “no-hair theorem” of the ART in connection with black holes.
Strong field tests
Pulsars are rapidly rotating neutron stars that constantly emit radio pulses during this rotation. Because of this, they can also be viewed as clocks, which allows very precise checks of their orbital movements. Observations of pulsars that are in orbit around other stars have all shown perihelion rotations that cannot be explained in the classic way, but only with GTR. For example, the Hulse-Taylor binary pulsar PSR 1913 + 16 (a pair of neutron stars, one of which is a pulsar) has an observed precession of over 4 ° per year. This precession was used to calculate the mass of the components.
Analogous to the emission of electromagnetic radiation by atoms and molecules, a mass distribution with a quadrupole moment or a higher type of vibration, or if it is asymmetrical and in rotation, can emit gravitational waves . These should travel at the speed of light. For example, planets in orbit around the sun lose energy via gravitational radiation, but this effect is so small that it is unlikely that it can be observed in the near future (the gravitational radiation of the earth amounts to approx. 200 watts ). This radiation could be detected indirectly with the Hulse-Taylor pulsar. Precise time measurements of the pulsars showed that their orbits only approximately correspond to Kepler's laws , because over time they move towards each other in a spiral, whereby they show an energy loss that is in close agreement with the predicted energy output by gravitational waves. So even though the waves have not been measured directly, it is necessary to consider their effects in order to explain the orbits. For this work, Hulse and Taylor received the Nobel Prize.
The double pulsar PSR J0737-3039 discovered in 2003 shows a perihelion rotation of 16.90 ° per year; In contrast to the Hulse-Taylor pulsar, both stars are pulsars, which allows precision measurements of both parts of the system. Due to the fact that the system can be observed almost directly "at the edge" ( inclination 90 °), and the very low transverse speed of the system from the point of view of the earth, J0737−3039 is by far best suited for tests of strong gravitational fields the ART. Several different effects have been observed including the decrease in orbit as in the Hulse-Taylor system. After observing the system for 2½ years, four independent tests of ART were possible, the most accurate (the Shapiro delay) confirming ART's predictions to within 0.05%.
Experiments to detect black holes are indirect in nature. They concern the effects of their extraordinarily strong gravitational fields on nearby stars, the formation of accretion disks , the deflection of light rays, gravitational time dilation and redshift as well as other effects. For more details, see: Black Hole Observation Methods .
Gravitational waves
A number of gravitational wave detectors have been set up to directly measure gravitational waves emitted by astronomical objects, such as when two neutron stars merge. Currently, the most accurate detector is the Laser Interferometer Gravitational-wave Observatory ( LIGO ), which has been in operation since 2002 and has since been expanded to become an Advanced LIGO detector. This was able to measure the first gravitational waves directly in September 2015. Future detectors with significantly improved precision are being developed or are being planned, such as the planned Mission Laser Interferometer Space Antenna (LISA) or the Einstein telescope . It is expected that Advanced-LIGO can observe gravitational wave events possibly every day.
Cosmological tests
Tests of ART on the largest possible cosmological scale are not nearly as compelling as, for example, solar system tests. The first of these tests was the prediction and discovery of the expansion of the universe. In 1922 Alexander Alexandrowitsch Friedmann found that the equations of the GTR contain non-stationary solutions (even in the presence of a cosmological constant ). In 1927, Georges Lemaître showed that static solutions of the equations of the GR, like Einstein's original solution, which are supposed to occur in the presence of a cosmological constant, are unstable and therefore do not exist. H. the universe must either expand or contract. Lemaître made the explicit prediction that the universe will expand. He also inferred a relationship between redshift and distance that came to be known as Hubble's law . The redshift actually discovered by Edwin Hubble (1929) and the associated expansion of the universe was regarded by many (even today) as a direct confirmation of the predictions of the GTR. This led to Einstein also agreeing with the solutions of Friedmann and Lemaître in 1931. However, in the 1930s, mainly through the work of Edward Arthur Milne , it was recognized that the linear relationship between redshift and distance derives more from the general assumption of uniformity and isotropy than from GTR specifically. Nevertheless, the then dramatic prediction of a non-static universe was by no means trivial and was mainly motivated by the GTR.
Some other cosmological tests are the search for gravitational waves generated during cosmic inflation , which could be observed in the polarization of the cosmic background radiation or with the planned space-based gravitational wave interferometer " Big Bang Observer " (BBO). Other tests at high redshift aim to limit the possibility of alternative theories of gravitation and to check the variation in the gravitational constant since primordial nucleosynthesis .
Individual evidence
Secondary sources
- N. Ashby: Relativity in the Global Positioning System . In: Living Reviews in Relativity . tape 6 , no. 1 , 2003 ( livingreviews.org [accessed June 23, 2011]).
- S. Chandrasekhar: The Role of General Relativity in Astronomy: Retrospect and Prospect . In: Journal of Astrophysics and Astronomy . tape 1 , no. 1 , 1980, p. 33-45 , doi : 10.1007 / BF02727948 ( ias.ac.in [PDF]).
- L. Iorio (Ed.): The Measurement of Gravitomagnetism: A Challenging Enterprise . Hauppage (New York) 2007, ISBN 1-60021-002-3 .
- Helge Kragh, Robert W. Smith: Who discovered the expanding universe . In: History of Science . tape 41 , 2003, p. 141-162 , bibcode : 2003HisSc..41..141K .
- Charles W. Misner, Kip S. Thorne, John Archibald Wheeler: Gravitation . Ed .: Freeman. San Francisco 1973, ISBN 978-0-7167-0344-0 .
- W. Pauli: Theory of Relativity . Ed .: Springer. Heidelberg 2000, ISBN 3-540-67312-1 , part 4. General theory of relativity.
- Slava G. Turyshev: Experimental Tests of General Relativity . In: Annual Review of Nuclear and Particle Systems . tape 58 , no. 1 , 2008, p. 207-248 , arxiv : 0806.1731 .
- CM Will : Theory and Experiment in Gravitational Physics . Ed .: University Press. Cambridge 1993, ISBN 0-521-43973-6 .
- CM Will: 100 years of relativity . In: A. Ashtekar (Ed.): World Scientific . Singapore 2005, ISBN 981-256-394-6 , What Einstein Right? Testing Relativity at the Centenary, p. 205-227 , arxiv : gr-qc / 0504086 .
- CM Will: The Confrontation between General Relativity and Experiment . In: Living Reviews in Relativity . tape 9 , no. 3 , 2006 ( livingreviews.org [accessed June 23, 2011]).
- CM Will: Resource Letter PTG-1: Precision Tests of Gravity . In: American Journal of Physics . tape 78 , no. 12 , 2010, p. 1240-1247 , arxiv : 1008.0296 .
Primary sources
- ↑ a b Albert Einstein: The basis of the general theory of relativity . In: Annals of Physics . tape 49 , 1916, pp. 769-782 ( uni-augsburg.de [PDF]).
- ↑ U. Le Verrier (1859), (in French), “Lettre de M. Le Verrier à M. Faye sur la théorie de Mercure et sur le mouvement du périhélie de cette planète” , Comptes rendus hebdomadaires des séances de l'Académie des sciences (Paris), vol. 49 (1859), pp. 379-383.
- ↑ Tai L. Chow,: Gravity, black holes, and the very early universe: an introduction to general relativity and cosmology . Ed .: Springer. 2008, ISBN 0-387-73629-8 , pp. 70 .
- ^ Richard Alfred Matzner: Dictionary of geophysics, astrophysics, and astronomy . Ed .: CRC Press. 2001, ISBN 0-8493-2891-8 , pp. 356 .
- ^ Weisberg et al .: The Relativistic Binary Pulsar B1913 + 16: Thirty Years of Observations and Analysis . In: Astronomical Society of the Pacific (Ed.): ASP Conference Series . 328 Binary Radio Pulsars. . San Francisco July 2005, p. 25 , arxiv : astro-ph / 0407149 , bibcode : 2005ASPC..328 ... 25W ( aspbooks.org ).
- ↑ Johann Georg von Soldner : About the deflection of a ray of light from its rectilinear movement, through the attraction of a cosmic body, which it passes close. In: Astronomical Yearbook for 1804 . 1804, p. 161-172 .
- ↑ Albert Einstein: About the influence of gravity on the spread of light . In: Annals of Physics . tape 340 , no. 10 , 1911, pp. 898-908 ( uni-augsburg.de [PDF]).
- ^ A b C. M. Will: The Confrontation between General Relativity and Experiment . In: Living Rev. Relativity . tape 9 , 2006, p. 39 ( livingreviews.org ).
- ^ FW Dyson, AS Eddington, C. Davidson: A determination of the deflection of light by the Sun's gravitational field, from observations made at the total eclipse of 29 May 1919 . In: Philos. Trans. Royal Soc. London . 220A, 1920, p. 291-333 .
- ^ Rosenthal-Schneider, Ilse: Reality and Scientific Truth. Detroit: Wayne State University Press, 1980. p. 74. See also Alice Calapric : The New Quotable Einstein. Princeton: Princeton University Press, 2005, p. 227.
- ↑ Daniel Kennefick, "Not Only Because of Theory: Dyson, Eddington and the Competing Myths of the 1919 Eclipse Expedition," Proceedings of the 7th Conference on the History of General Relativity , Tenerife, 2005; arxiv : 0709.0685
- ↑ Philip Ball: Arthur Eddington was innocent! In: news @ nature. 2007, doi: 10.1038 / news070903-20 .
- ↑ a b Daniel Kennefick, "Testing relativity from the 1919 eclipse - a question of bias," Physics Today, March 2009, pp 37-42.
- ↑ Albert Einstein: About the principle of relativity and the conclusions drawn from it . In: Yearbook of radioactivity and electronics . tape 4 , 1908, pp. 411-462 ( soso.ch [PDF]).
- ^ RV Pound, GA Rebka Jr .: Gravitational Red-Shift in Nuclear Resonance . In: Physical Review Letters . tape 3 , no. 9 , November 1, 1959, p. 439–441 , doi : 10.1103 / PhysRevLett.3.439 , bibcode : 1959PhRvL ... 3..439P .
- ^ RV Pound, GA Rebka Jr .: Apparent weight of photons . In: Physical Review Letters . tape 4 , no. 7 , April 1, 1960, pp. 337–341 , doi : 10.1103 / PhysRevLett.4.337 , bibcode : 1960PhRvL ... 4..337P .
- ↑ RH Dicke: New Research on Old Gravitation: Are the observed physical constants independent of the position, epoch, and velocity of the laboratory? In: Science . tape 129 , no. 3349 , March 6, 1959, p. 621-624 , doi : 10.1126 / science.129.3349.621 , PMID 17735811 , bibcode : 1959Sci ... 129..621D .
- ↑ RH Dicke: Mach's Principle and Equivalence . In: Academic Press (Ed.): Evidence for gravitational theories . 1962.
- ^ Leonard Isaac Schiff : On Experimental Tests of the General Theory of Relativity . In: American Journal of Physics . tape 28 , no. 4 , April 1, 1960, p. 340–343 , doi : 10.1119 / 1.1935800 , bibcode : 1960AmJPh..28..340S .
- ^ CH Brans, RH Dicke: Mach's Principle and a Relativistic Theory of Gravitation . In: Physical Review . tape 124 , no. 3 , November 1, 1961, pp. 925-935 , doi : 10.1103 / PhysRev.124.925 , bibcode : 1961PhRv..124..925B .
- ↑ EB Fomalont, SM Kopeikin, G. Lanyi, J. Benson: Progress in Measurements of the Gravitational Bending of Radio Waves Using the VLBA . In: Astrophysical Journal . tape 699 , no. 2 , July 2009, p. 1395-1402 , doi : 10.1088 / 0004-637X / 699/2/1395 , bibcode : 2009ApJ ... 699.1395F .
- ↑ SS Shapiro, JL Davis, DE Lebach, JS Gregory: Measurement of the solar gravitational deflection of radio waves using geodetic very-long-baseline interferometry data, 1979-1999 . In: American Physical Society (Ed.): Physical Review Letters . tape 92 , no. 121101 , March 26, 2004, p. 121101 , doi : 10.1103 / PhysRevLett.92.121101 , PMID 15089661 , bibcode : 2004PhRvL..92l1101S .
- ↑ M. Froeschlé, F. Mignard and F. Arenou, “ Determination of the PPN parameter γ with the Hipparcos data ” Hipparcos Venice '97, ESA-SP-402 (1997).
- ^ II Shapiro: Fourth test of general relativity . In: Physical Review Letters . tape 13 , no. 26 , December 28, 1964, pp. 789–791 , doi : 10.1103 / PhysRevLett.13.789 , bibcode : 1964PhRvL..13..789S .
- ^ II Shapiro, ME Ash, RP Ingalls, WB Smith, DB Campbell, RB Dyce, RF Jurgens, and Gordon Pettengill : Fourth Test of General Relativity: New Radar Result . In: Physical Review Letters . tape 26 , no. 18 , May 3, 1971, pp. 1132–1135 , doi : 10.1103 / PhysRevLett.26.1132 , bibcode : 1971PhRvL..26.1132S .
- ↑ B. Bertotti, Iess L., P. Tortora: A test of general relativity using radio links with the Cassini spacecraft . In: Nature . tape 425 , 2003, p. 374 , doi : 10.1038 / nature01997 , bibcode : 2003Natur.425..374B .
- ↑ SM Kopeikin et al .: Gravimagnetic effect of the barycentric motion of the Sun and determination of the post-Newtonian parameter γ in the Cassini experiment . In: Physics Letters A . tape 367 , 2007, p. 76 , doi : 10.1016 / j.physleta.2007.03.036 , arxiv : gr-qc / 0604060 , bibcode : 2007PhLA..367..276K .
- ↑ EB Fomalont, SM Kopeikin: The Measurement of the Light Deflection from Jupiter: Experimental Results . In: Astrophysical Journal . tape 598 , no. 1 , November 2003, p. 704–711 , doi : 10.1086 / 378785 , arxiv : astro-ph / 0302294 , bibcode : 2003ApJ ... 598..704F .
- ↑ EB Fomalont, SM Kopeikin, D. Jones, M. Honma, O. Titov: Recent VLBA / VERA / IVS tests of general relativity . In: Proceedings of the International Astronomical Union, IAU Symposium . tape 261 , S261, January 2010, pp. 291–295 , doi : 10.1017 / S1743921309990536 , bibcode : 2010IAUS..261..291F .
- ^ K. Nordtvedt Jr .: Equivalence Principle for Massive Bodies. II. Theory . In: Physical Review . tape 169 , no. 5 , May 25, 1968, pp. 1017-1025 , doi : 10.1103 / PhysRev.169.1017 , bibcode : 1968PhRv..169.1017N .
- ^ K. Nordtvedt Jr .: Testing Relativity with Laser Ranging to the Moon . In: Physical Review . tape 170 , no. 5 , June 25, 1968, p. 1186–1187 , doi : 10.1103 / PhysRev.170.1186 , bibcode : 1968PhRv..170.1186N .
- ↑ a b J. G. Williams; Slava G. Turyshev, Dale H. Boggs: Progress in Lunar Laser Ranging Tests of Relativistic Gravity . In: Physical Review Letters . tape 93 , no. 5 , December 29, 2004, p. 1017-1025 , doi : 10.1103 / PhysRevLett.93.261101 , arxiv : gr-qc / 0411113 , bibcode : 2004PhRvL..93z1101W .
- ↑ RFC Vessot; MW Levine, EM Mattison, EL Blomberg, TE Hoffman, GU Nystrom, BF Farrel, R. Decher, PB Eby, CR Baugher, JW Watts, DL Teuber and FD Wills: Test of Relativistic Gravitation with a Space-Borne Hydrogen Maser . In: Physical Review Letters . tape 45 , no. 26 , December 29, 1980, pp. 2081–2084 , doi : 10.1103 / PhysRevLett.45.2081 , bibcode : 1980PhRvL..45.2081V .
- ^ N. Ashby, 2003
- ^ J. Hafele, R. Keating: Around the world atomic clocks: predicted relativistic time gains . In: Science . tape 177 , no. 4044 , July 14, 1972, p. 166-168 , doi : 10.1126 / science.177.4044.166 , PMID 17779917 , bibcode : 1972Sci ... 177..166H .
- ^ J. Hafele, R. Keating: Around the world atomic clocks: observed relativistic time gains . In: Science . tape 177 , no. 4044 , July 14, 1972, p. 168–170 , doi : 10.1126 / science.177.4044.168 , PMID 17779918 , bibcode : 1972Sci ... 177..168H .
- ↑ CW Chou, DB Hume, T. Rosenband, DJ Wineland: Optical Clocks and Relativity . In: Science . tape 329 , no. 5999 , 2010, pp. 1630–1633 , doi : 10.1126 / science.1192720 , bibcode : 2010Sci ... 329.1630C .
- ^ I. Ciufolini, EC Pavlis: A confirmation of the general relativistic prediction of the Lense-Thirring effect . In: Nature . tape 431 , no. 7011 , 2004, p. 958–960 , doi : 10.1038 / nature03007 , PMID 15496915 , bibcode : 2004Natur.431..958C .
- ↑ Lorenzo Iorio : Conservative evaluation of the uncertainty in the LAGEOS-LAGEOS II Lense-Thirring test . In: Central European Journal of Physics . tape 8 , no. 1 , 2009, p. 25 , doi : 10.2478 / s11534-009-0060-6 , bibcode : 2010CEJPh ... 8 ... 25I .
- ↑ Lorenzo Iorio: COMMENTS, REPLIES AND NOTES: A note on the evidence of the gravitomagnetic field of Mars . In: Classical Quantum Gravity . tape 23 , no. 17 , 2006, p. 5451-5454 , doi : 10.1088 / 0264-9381 / 23/17 / N01 , arxiv : gr-qc / 0606092 , bibcode : 2006CQGra..23.5451I .
- ^ K. Krogh: Comment on 'Evidence of the gravitomagnetic field of Mars' . In: Classical Quantum Gravity . tape 24 , no. 22 , 2007, p. 5709–5715 , doi : 10.1088 / 0264-9381 / 24/22 / N01 , bibcode : 2007CQGra..24.5709K .
- ↑ Lorenzo Iorio: On the Lense-Thirring test with the Mars Global Surveyor in the gravitational field of Mars . In: Central European Journal of Physics . 2009, doi : 10.2478 / s11534-009-0117-6 , arxiv : gr-qc / 0701146 , bibcode : 2010CEJPh ... 8..509I .
- ↑ Everitt et al .: Gravity Probe B: Final Results of a Space Experiment to Test General Relativity . In: Physical Review Letters . tape 106 , no. 22 , 2011, p. 221101 , doi : 10.1103 / PhysRevLett.106.221101 , arxiv : 1105.3456 .
- ↑ Lorenzo Iorio: Advances in the measurement of the Lense-Thirring effect with Planetary Motions in the Field of the Sun . In: Scholarly Research Exchange . tape 2008 , 2008, 105235, pp. 1 , doi : 10.3814 / 2008/105235 , bibcode : 2008ScReE2008.5235I .
- ↑ David Merritt, T. Alexander, S. Mikkola, C. Will: Testing Properties of the Galactic Center Black Hole Using Stellar Orbits . In: The Physical Review D . tape 81 , no. 6 , 2010, p. 062002 , doi : 10.1103 / PhysRevD.81.062002 , bibcode : 2010PhRvD..81f2002M .
- ^ Clifford Will : Testing the General Relativistic “No-Hair” Theorems Using the Galactic Center Black Hole Sagittarius A * . In: Astrophysical Journal Letters . tape 674 , no. 1 , 2008, p. L25 – L28 , doi : 10.1086 / 528847 , bibcode : 2008ApJ ... 674L..25W .
- ^ M. Kramer et al .: Tests of general relativity from timing the double pulsar . In: Science . tape 314 , no. 5796 , 2006, pp. 97–102 , doi : 10.1126 / science.1132305 , PMID 16973838 , arxiv : astro-ph / 0609417 , bibcode : 2006Sci ... 314 ... 97K .
- ^ BP Abbott et al .: Observation of Gravitational Waves from a Binary Black Hole Merger (PDF) , LIGO Scientific Collaboration and Virgo Collaboration, Physical Review Letters, February 11, 2016
- ↑ PJE Peebles: Testing general relativity on the scales of cosmology . In: World Scientific Publishing Co. Pte. Ltd. December 2004, doi : 10.1142 / 9789812701688_0010 , arxiv : astro-ph / 0410284 , bibcode : 2005grg..conf..106P .
- ↑ G. Lemaître: Expansion of the universe, A homogeneous universe of constant mass and increasing radius accounting for the radial velocity of extra-galactic nebulæ . In: Monthly Notices of the Royal Astronomical Society . tape 91 , 1931, p. 483-490 , bibcode : 1931MNRAS..91..483L . (Translation from Un Univers homogène de masse constante et de rayon croissant rendant compte de la vitesse radiale des nébuleuses extra-galactiques , 1927).
- ↑ Kragh, 2003, p. 152
- ↑ Kragh, 2003, p. 153.
- ^ Pauli, 1958, pp. 219-220
- ^ Edward Arthur Milne: Relativity, gravitation and world-structure . Ed .: Clarendon Press. Oxford 1935.
- ↑ Chandrasekhar, 1980, p. 37