Crassulacean acid metabolism
The Crassulacean acid metabolism (short CAM of Crassulacean Acid Metabolism ) is a special metabolism of various plants . While most plants uptake and fixation of carbon dioxide to carry the day, these operations are separated in time in plants with CAM. The carbon dioxide required for photosynthesis is absorbed at night and chemically stored in the cell's vacuoles in the form of malic acid . The following day, the carbon dioxide is released again from the malic acid and used to build up carbohydrates in the Calvin cycle . Because of the daily build-up and breakdown of this dicarboxylic acid and the associated amount of protons alternating between day and night, this metabolism is also known as the diurnal acid rhythm (Latin diurnus = "daily").
The advantage of the CAM mechanism is that the plant can keep its stomata closed during the (hot) hours of the day , which means that it loses significantly less water through transpiration and still has sufficient amounts of carbon dioxide available in the Calvin cycle. In addition, CAM is a beneficial response to low levels of carbon dioxide, as it can also be seen in submerged freshwater plants, for example. So far, this type of metabolism has only been demonstrated in tissue containing chlorophyll .
The Crassulaceae acid metabolism is named after the thick-leaf family ( Crassulaceae ) in which it was first discovered.
history
Certain aspects of the Crassulacean acid metabolism were noticed as early as the beginning of the 19th century. The Swiss naturalist de Saussure observed in 1804 that the branches of the opuntia produce oxygen in the light even when there is no carbon dioxide in the air; this is not the case with C 3 plants. He concluded from this that the plant consumed its own substances while releasing CO 2 . In 1813 Benjamin Heyne discovered the diurnal acid rhythm in the Goethe plant through a self-experiment: He noticed that the leaves tasted extremely sour in the morning, but had a herbal taste in the afternoon. Heyne reported this observation to the Linnaeus Society and later published these findings.
The term Crassulacean Acid Metabolism was first used in January 1947 by Meirion Thomas (1894-1977) at a lecture before the Society for Experimental Biology . In 1949 the term became part of the fourth edition of his standard work Plant Physiology . In the middle of the 20th century, the elucidation of the Crassulacean acid metabolism was mainly through the work of Walter Daniel Bonner (1878-1956) and his son James Frederick Bonner (1910-1996), Meirion Thomas, Thomas Archibald Bennet-Clark (1903-1975 ) and other researchers.
mechanism
Differentiated from C 3 and C 4 plants
All green plants photosynthesize to build up carbohydrates . In the " dark reaction ", carbon dioxide (CO 2 ) is fixed and built up into carbohydrates. Most plants ( C 3 plants ) operate a mechanism described as C 3 metabolism, in which carbon dioxide passively passes through the stomata into the cells and is fixed as a substrate during the day in the Calvin cycle. An adaptation of this mechanism can be found in C 4 plants , which actively increase the CO 2 concentration for the fixation, thus consuming energy . Here, there is a spatial separation (two cell types, mesophyll cells and bundle sheath cells ) for the pre-fixation and metabolization of carbon dioxide. This allows the plants to partially close their stomata because, unlike C 3 plants, they are not restricted by the simple diffusion of carbon dioxide into the cells. When the stomata are partially closed, it also reduces the outflow of water from the plant. Therefore, C 4 plants are found preferentially in dry and sunny places. The CO 2 fixation in the Calvin cycle , however, corresponds to that of C 3 plants.
In order to survive in arid regions, have CAM plants mechanisms, the steps of CO 2 fixation from those of the Calvin cycle time to separate. This allows the stomata to remain closed during the heat of the day to minimize water loss. In the cooler night, they are then opened for CO 2 absorption. While carbon dioxide is fixed in malate at night and stored in the vacuoles, it is released the following day and converted by RuBisCO , the key enzyme of the “dark reaction”, analogous to a C 3 plant. These biochemical reactions take place in a cell.

CA α-carbonic anhydrase
CC Calvin cycle
PEP phosphoenolpyruvate
PEPC phosphoenolpyruvate carboxylase
PEPCK phosphoenolpyruvate carboxykinase
MDH malate dehydrogenase
ME malate dehydrogenase (decarboxylating) = malate enzyme
PPDK pyruvate phosphate dikinase
Carbon dioxide fixation at night
During the night, CO 2 is fixed to phosphoenolpyruvate (PEP) by a cytosolic PEP carboxylase (PEPC, EC 4.1.1.31 ), similar to that of C 4 plants. The enzyme condenses hydrogen carbonate (HCO 3 - ) on PEP. The required PEP is usually provided by breaking down starch ; other plant species use soluble sugars such as glucose , sucrose or fructans for this . CAM plants must be able to provide large amounts of PEP - enough that an after-filtration of carbon dioxide can be fixed. For this purpose, the starch store and / or the supply of soluble sugars (in the vacuole) is replenished during the day, which are then able to deliver PEP at night. Therefore, PEP is a limiting factor for CAM, as it limits the amount of CO 2 to be fixed at night . In contrast to C 3 plants, starch degradation in chloroplasts ends at the level of glucose-6-phosphate , which is transported into the cytosol and degraded to PEP with ATP gain. In addition to providing additional energy, glucose-6-phosphate also activates PEPC.
Although CO 2 is in equilibrium with HCO 3 - in aqueous solutions, this conversion takes place only slowly. A carbonic anhydrase (CA, EC 4.2.1.1 ) accelerates the establishment of the equilibrium in favor of HCO 3 - , which is then converted to oxaloacetate (OA) by the PEPC . The OA formed during the reaction is reduced to L - malate by the likewise cytosolic NAD malate dehydrogenase (MDH, EC 1.1.1.37 ) . In the process, NADH is oxidized to NAD + .
The malate is transported into the vacuole and stored there. The vacuoles of CAM plants are very large compared to other species and can build up a comparatively high concentration of malate (up to 0.2 M ), which overall contributes to a high storage capacity. The deposition of malate in the vacuoles also keeps the pH value in the cytosol constant.
In order to transport malate into the vacuole against the concentration gradient , two protons per malate are brought into the vacuole while consuming ATP . This is catalyzed by a type V ATPase located on the membrane on the tonoplast . Through a secondary, active transport, malate follows the protons through a dicarboxylate transporter. The storage takes place in the protonated form of the malate, the malic acid , since in the very acidic conditions the malic acid is predominantly in undissociated form. This also has the advantage of a lower osmotic value as a result. In the case of the guard cell vacuoles , for example, potassium malate is stored in the vacuole, which causes a comparatively higher osmotic pressure. While the pH value of the vacuole of many C 3 and C 4 plants is 5.5, that of CAM plants can reach pH values of 3.0 at night due to the increased acid concentration. Therefore, the leaves of these plants taste very sour in the morning.
Release of carbon dioxide during the day
During the day, this path is formally reversed and the carbon dioxide is released again. The stored malate is probably transported from the vacuole into the cytosol via a carrier- mediated transport, in which two protons are also transported. It is possible that malic acid can also diffuse directly through the membrane as an uncharged particle. During the day, the pH value in the vacoule therefore rises again, reaching values of 7.5–8.0. This process is also known as acidification .
As with C 4 plants, the malate is broken down and carbon dioxide released in different ways, depending on the species, which reflects the independent development of the CAM in different taxa. The usual routes are the NADP-dependent malate enzyme (in cactus plants, agave plants), the NAD-dependent malate enzyme (in thick-leaf plants) and phosphoenolpyruvate carboxykinase (e.g. in bromeliads, lily plants, Asclepiadaceae).
- In plants with the NADP-dependent malate enzyme (NADP-ME, EC 1.1.1.40 ), the L -malate transported from the vacuole is decarboxylated to pyruvate . These reactions take place in the chloroplast . In doing so, NADP + is reduced to NADPH.
- Some plants have a mitochondrial , NAD-dependent malate enzyme (NAD-ME, EC 1.1.1.39 ), which uses NAD + instead of NADP + as a cofactor. The reactions take place analogously to the NADP-ME described above. However, pyruvate is not returned directly to the cytosol in the mitochondrion, but is initially transported into the chloroplast via the intermediate stage L - alanine . There it is built up into PEP and later brought into the cytosol. The amination to alanine and its reverse reaction is catalyzed by an alanine transaminase ( EC 2.6.1.2 ).
- CAM plants of the PEP carboxykinase type have two cycles. In addition to the one NAD-ME circuit, these also have a cytosolic PEP carboxykinase (PEPCK; EC 4.1.1.49 ). For this purpose, L -malate is first oxidized by an NAD-malate-DH (MDH, EC 1.1.1.37 ) to oxaloacetate while consuming NAD + . Oxaloacetate is then decarboxylated directly to PEP by the PEPCK, this takes place with the consumption of ATP.
The carbon dioxide released by the above-mentioned reactions is introduced into the Calvin cycle and built up to 3-phosphoglycerate , which leads to the further formation of monosaccharides ( D - glucose , D - fructose ) or polysaccharides (sucrose). The pyruvate formed during the decarboxylation reactions is converted to PEP by a pyruvate phosphate dikinase (PPDK, EC 2.7.9.1 ) with consumption of ATP. PEP is finally built up into starch, sucrose or fructan in the course of gluconeogenesis .
Since the majority of the carbohydrates formed are used to replenish the starch stocks / soluble sugars consumed the night before, the gain from CAM photosynthesis is low. In addition, the extent of malate storage is limited by the size of the vacuole. As a result, the increase in biomass is limited.
The build-up and breakdown of the malate results in strong changes in the pH value from pH 7.5 during the day to pH 3 at night. Because of this alternation of day and night, one speaks of a diurnal acid rhythm. This rhythm was first detected in 1984 in the breeding leaf Kalanchoe daigremontiana .
Forms of the CAM
CAM can be classified into different types. A distinction is made between when the stomata are opened. In addition, you can use the amount of malic acid formed during the night and the CO 2 uptake for the classification.
Obligatory or constitutive CAM
The metabolic mechanism of obligate CAM plants was presented in detail above. These always open their stomata during the night, during which 99% of the CO 2 fixation takes place. Depending on the strength of the CO 2 uptake and subsequent malic acid formation, a distinction is made between strongly and weakly obligate CAM. Typical representatives of the obligatory CAM can be found, for example, with the thick-leaf plants ( Kalanchoe daigremontiana ) and the cactus plants ( Opuntia basilaris and Opuntia ficus-indica ). In contrast to the other CAM types, these plants always remain in that CAM mode, regardless of the type and strength of abiotic stress. However, it has been observed that very young seedlings of obligatory CAM plants, such as the Goethe plant , the Opuntia ficus-indica and Kalanchoe daigremontiana , can still have a C 3 metabolism.
CAM in these plants is divided into four phases. In phase I at the beginning of the night, the stomata are opened and carbon dioxide is fixed by PEPC (see above ). At the end of phase I, the stomata close. Some plants then enter phase II , in which the stomata reopen in the early, not dark hours of the morning. This is how atmospheric carbon dioxide gets into the plant, which is fixed in particular by the PEPC and additionally by RuBisCO. Light and low cytosolic CO 2 levels are a signal to reopen the stomata. Later the CAM plant enters phase III , the stomata remain closed (see above ). Here, malate is decarboxylated in the ways described above, so that the cytosolic CO 2 concentration increases sharply. This is a signal to close the stomata. In the course of the afternoon, malate is consumed, so that the cytosolic CO 2 concentration drops again. Since there is still light, the stomata are opened again in phase II. This is phase IV . This is the phase in which photorespiration is highest. Finally, at nightfall, the plant returns to phase I.
Phase II and IV give the CAM plant additional CO 2 uptake, which is associated with faster growth. However, this depends on the availability of water, so that phase I and III dominate in many obligatory CAM plants.
Optional or inducible CAM (C 3 -CAM)
It has been observed that some plants can have both a CO 2 fixation in the sense of a C 3 plant and a CAM plant. Representatives are often found in the ice plant family , thick-leaf family ( sedum plant ), purslane family ( Calandrinia species), grapevine family and many species of the genus Clusia . The best studied plant with facultative CAM is the ice herb. Other representatives are bacon tree or agave deserti . These plants are found in semi-arid regions, on rocky ground, on tree branches and generally in habitats with water scarcity.
In the non-induced status, the CO 2 uptake proceeds analogously to a normal C 3 plant during the day with the stomata open. No malic acid is formed during the night either. After induction, however, there is nocturnal CO 2 fixation and malic acid formation, analogous to a weak obligate CAM plant. The induction is triggered by drought (during periods of drought), salinity, high amounts of light, nitrogen and phosphate deficiency. Sometimes it also depends on the age of the plant. If some seedlings still have insufficiently large vacuoles, photosynthesis is carried out in the sense of a C 3 plant. Only as it grows older does the plant switch to CAM. In Kalanchoe blossfeldiana, photoperiodism induces the change between C 3 and CAM.
The change between CAM and C 3 -CO 2 fixation is quick and usually reversible. In this way, it is possible to switch to CAM within a day if the temperature rises and the water supply deteriorates. Sometimes a part of the plant also operates CAM, while - at least with a good water supply - another part carries out C 3 photosynthesis. This is the case, for example, with Frerea indica , in which the succulent stem has CAM, whereas the leaves have a C 3 metabolism. In tropical Clusia species it can even happen that one leaf carries out C 3 metabolism, but an opposite one carries out CAM. Some optional CAM plants can also have a C 3 metabolism throughout their life without ever switching to CAM. In Clusia cylindrica , for example, the C 3 metabolism is the main path for CO 2 fixation.
In the case of the ice herb , an annual salt plant , the change from C 3 to CAM is irreversible under natural conditions. The plant grows in the Mediterranean area , among other places , and switches to CAM when there is a shortage of water and salt stress. The latter is a response to the associated water scarcity, not so much because of the increased number of ions. It has also been found that the switch to CAM, even without these two stressors, occurs in older plants. Salt or water stress only accelerate a programmed development in ice herb, which inevitably leads to CAM. The light intensity and quality can also induce CAM in the ice herb. Under controlled experimental conditions, however, a change from CAM back to C 3 metabolism could be observed in younger plants .
In the family of portulacaceae also a change of the CO 2 fixation of C 4 has been observed to be the CAM. To set Portulaca grandiflora , summer purslane , Portulaca Mundula , but also Peperomia camptotricha with water shortages on their metabolism to CAM. However, the C 4 metabolism and CAM do not take place in one and the same cell.
Plants Showing Optional CAM Bacon tree Boucerosia frerea Ice herb Purslane florets
CAM idle ( idling )
In plants suffering from extreme water scarcity, the stomata are closed both during the day and at night in order to keep water loss as low as possible. The carbon dioxide released during breathing is refixed, so that the nocturnal formation of malic acid is low. During this stressful situation the photosystems remain intact, the plant “waits” for better conditions and is thus in a kind of “idle state”. Nevertheless, a diurnal acid rhythm also takes place here. This was observed for the first time in Opuntia basilaris .
There is no net carbon uptake, so plants cannot grow in this state, but can survive.
CAM "change" ( cycling )
In this mode, also known as “almost C 3 ”, the stomata remain closed at night. The carbon dioxide released by breathing is fixed, which means that small amounts of malic acid are stored in the vacuoles. During the day the CO 2 fixation takes place in the sense of a C 3 photosynthesis. In addition, the malate formed during the night is decarboxylated. Therefore, despite the open stomata, the plants show a diurnal acid rhythm during the day. Plants with such a CAM type are found in locations where optional CAM plants also grow. Representatives of this CAM type are Peperomia camptotricha or Talinum calycinum , the latter grows on dry, rocky soil. In Isoetes howellii , a water plant, CAM cycling particularly pronounced. The epiphyte Codonanthe crassifolia operates CAM cycling when there is a good water supply, but switches to CAM idling when it is dry.
regulation
A fixation of the carbon dioxide by the PEP carboxylase (PEPC) during the day is prevented by an allosteric inhibition of the enzyme: The malate flowing out of the vacuole and a low pH value - daytime conditions - effectively inhibit PEPC. This is to prevent carbon dioxide as a substrate from being unnecessarily consumed for a reaction other than RuBisCO. Also, L - aspartate inhibits the PEPC, whereas glucose-6-phosphate and Triosephosphate activate the enzyme. Two genes code for PEPC, Pepc1 and Pepc2 . Its expression is influenced by dryness, abscisic acid and high salinity.
However, the enzyme activity is - as with C 4 plants - additionally regulated by reversible phosphorylation on a serine residue . The dephosphorylated form of PEPC is 10 times more sensitive to malate than the phosphorylated and active form at night. The phosphorylation is mediated by a kinase , the PEP carboxylase kinase (PPCK1). This is subject to a strict, circadian rhythm of its gene expression and, due to its rapid build-up and breakdown, also its overall activity. Expression peaks in the middle of the night and then drops to a minimum level during the day. This does not depend on the light.
In contrast , the phosphatase (type 2A) responsible for dephosphorylation does not show any such rhythm in its activity.
During phase II of the CAM (see above), PEPC is still phosphorylated and active because malate is stored in the vacuole. During phase IV, malate is largely consumed and can no longer inhibit PEPC.
Occurrence
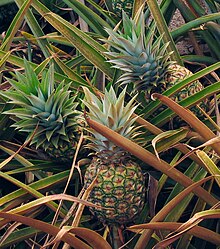
The CAM mechanism has been demonstrated in at least 35 plant families and 343 genera . It is estimated that the metabolic pathway occurs in over 16,000 species , which corresponds to 6–8% of all vascular plants . In addition to the thick-leaf family from which it is named (e.g. the Goethe plant ), there are other families with CAM species:
- Affodillaceae , z. B. the real aloe
- Agave family
- Bromeliads , e.g. B. the pineapple
- Clusiaceae
- Didiereaceae
- some epiphytic ferns , such as Pyrrosia piloselloides and Pyrrosia longifolia , are CAM plants.
- Dog poison plants
- Cactus plants , e.g. B. Echinocactus grusonii
- Daisy family
- Lily family
- Midday flowers , e.g. B. the living stones
- Orchids , e.g. B. the vanilla and phalaenopsis
- Purslane family
- Grapevines
- Spurge family .
Known representatives are the pineapple , Kalanchoe , but also the Central European Fettenhennen - and Hauswurz TYPES ( Sedum and Sempervivum ). In the plant kingdom, CAM is not phylogenetically grouped in the same way as in C 4 photosynthesis, but a repeated origin of CAM can be shown in some lineages . CAM is particularly well represented in cactus plants, milkweed plants and thick-leaf plants (eudicotyledons) or bromeliads, orchids and asparagus / agave plants (monocotyledons).
Many species occur in periodically dry areas, especially in deserts and savannas of the tropics and subtropics . However, savannahs are not typical habitats for these plants. There, CAM plants are often overgrown by C 3 or C 4 grasses. CAM plants are also often found in coastal regions or near salt lakes , although they usually avoid salt and do not really belong to the salt plants . CAM plants also grow in residual gas . These are coastal subtropical or tropical wet rainforests with sandy and nutrient-poor soil, which can be found in Brazil . Lack of water is also characteristic of the locations of epiphytes in rainforests. Therefore, many epiphytes are CAM plants, according to estimates between 50 and 60% of all epiphytic orchids and bromeliads. Some freshwater plants also show CAM, such as the thick water leaf and the European strandling , and some species of the genus Brachsenkräuter ( Isoëtes ) such as the sea bream . Finally, CAM plants can also be found on island mountains and at great heights, e.g. B. in the Alps ( mountain houseleek ) or in the Andes ( Echeveria quitensis , Oroya peruviana ). Some cacti such as Opuntia streptacantha or Opuntia humifusa can tolerate temperatures below freezing point. In general, however, low daytime temperatures hinder the release of malate from the vacuole (reduced viscosity and membrane permeability) and thus the CAM metabolism.
It is worth mentioning that Australia's natural flora is home to relatively few CAM plants. In 2016, 120 CAM plants were counted, most of which belong to the epiphytes, seven are terrestrial land plants. Large stem succulents are completely absent.
morphology
There is no typical appearance of a CAM plant as they are very different morphologically . However, all CAM plants are characterized by a more or less pronounced succulence and have particularly large vacuoles . The latter is also known as “succulence at the cellular level”. The vacuoles can fill up to 98% of the cell. This generally enables the storage of water and in particular of malate for the CAM cycle. The more succulent a CAM plant, the greater the CO 2 uptake at night. In terms of water conservation, these plants also have a thick cuticle , smaller stomata, and a small surface / volume ratio. Due to the large cells, the intercellular air space is very small, which limits the diffusion of released carbon dioxide during the day.
Morphotypes of CAM plants can be classified differently. There are leaf succulents such as Kalancho species. Sometimes these have a non-green, water-storing tissue that lies around, under or in the middle of the mesophyll . In addition to leaf succulents, you can also find stem succulents, which are particularly characteristic of arid regions and deserts. These have a central, water-storing tissue in the middle of the trunk. Epiphytes form their own physiognomy . In addition to strangler and climbing plants, there are also epiphytic, thornless cacti. CAM plants are often also rosette plants with specializations such as B. the Phytotelmata of some bromeliads.
A special feature of the genus Clusia represents dicotyledonous tree-forming species , able to operate the CAM. These are currently the only known trees with CAM. Due to the photosynthetic plasticity, the genus Clusia has established itself in various ecosystems. Clusia species themselves differ widely in the characteristics of CAM (see also section Forms of CAM ). On the other hand, some Clusia species, such as Clusia multiflora and Clusia cretosa , are pure C 3 plants.
The Joshua palm lily also forms a "tree", but this is an example of secondary growth in monocots and should not be confused with secondary growth in dicotyledons.
Importance of the CAM
The ecological advantage of the CAM is that the CO 2 absorption and thus the opening of the stomata takes place at night, while they remain closed during the day, thus greatly reducing water loss. This results in an extremely low water requirement per dry weight of 18–100 ml · g −1 during the day, in contrast to 450–950 ml · g −1 for C 3 plants. This means that the water requirement is only 5 to 10% compared to that of C 3 plants. CAM is therefore a successful strategy in order to be able to carry out photosynthesis despite the severe water shortage. This is also by the water use efficiency ( water-use efficiency brought WUE) expressed. The higher this efficiency, the lower the carbon gain (see table below). In the case of particularly severe dehydration, the stomata remain closed even at night and only the carbon dioxide released by breathing is fixed ( CAM idle ). This enables the plants to survive long periods of extreme drought. In addition to saving water losses during the day, a CAM plant experiences additional water gain at night. The storage of malic acid creates a high osmotic pressure in the vacuoles. As a result, water is absorbed from the ambient air, especially after being baptized at night.
The increased energy requirement for carbon dioxide assimilation compared to the C 3 plants is hardly significant in view of the high solar radiation at most of the locations of the CAM plants. In addition, the light saturation of photosynthesis is usually only reached at higher light intensities than with C 3 plants. For each fixed molecule of carbon dioxide, three molecules of ATP and two molecules of NADPH are required in the C 3 metabolism (without loss of photorespiration). In the case of CAM, these values are 4.8 to 5.9 ATP and 3.2 to 3.9 mol NADPH. While light is usually never a limiting factor, it could be a problem with epiphytes . During the rainy season in rainforests, the amount of light incident can become a problem due to the high humidity and evaporation. Individuals of the bromeliad family Bromelia humilis , for example, showed less growth in the shade than plants of the same species that grow in comparatively lighter locations.
To protect the photosystem, CAM plants have all mechanisms from C 3 plants ( zeaxanthin , xanthophyll cycle , D1 protein turnover, possibly photorespiration). CAM plants usually show hardly any photorespiration, as the cytosolic CO 2 concentration is very high due to the release of carbon dioxide from stored malate . Compared to C 3 plants, CAM plants also react less to oxidative stress caused by ozone (O 3 ) and sulfur dioxide (SO 2 ).
Finally, CAM is a CO 2 enrichment pump. This enables aquatic CAM plants to gain CO 2 at night. This allows them to assert themselves against other, non-CAM aquatic plants, which remove a lot of carbon dioxide from the water during the day. Sometimes the CO 2 content in the water is low even without competition from other plants if the water has a low pH value. For example, Isoetes howellii has advantages in this environment through CAM. During the night, aquatic CAM plants also take advantage of the fact that carbon dioxide builds up in the water through the respiration of microbes, animals and other (C 3 ) plants. In many bream herbs, the roots also absorb inorganic carbon and transfer it as CO 2 into the shoot. Bromeliads in extremely humid tropical cloud forests also benefit from CO 2 enrichment. During the rainy season or when there is heavy fog, the gas exchange on leaf surfaces is severely restricted. This gives CAM bromeliads an advantage over C 3 bromeliads, especially since the water-saving CAM mechanisms are advantageous during the dry season.
Another advantage of this ATP-driven CO 2 pump is the increased concentration of carbon dioxide during the day. This means that less RuBisCO is required, while the enzyme in C 3 plants makes up approx. 50% of the total soluble protein amount in the leaf. It was discussed that this gives CAM plants an advantage over C 3 plants in low-nitrogen soils, as they have better nitrogen-use efficiency (NUE). Nonetheless, it must be countered that even C 3 plants have corresponding adaptations in nitrogen-poor soils, so that CAM plants do not automatically have an advantage.
CAM plants always show a lower growth rate than C 4 or C 3 plants due to the limited storage capacity of the vacuoles. However, CAM plants grow in habitats in which survival is primarily more important than the gain in biomass.
Isotope Discrimination
As with isotope discrimination in C 4 plants , the PEP carboxylase in CAM plants does not discriminate against 13 C as strongly as RuBisCO. Therefore, their isotope ratio (δ- 13 C) corresponds to that of C 4 plants with CO 2 dark fixation , but that of C 3 plants with light fixation of external CO 2 . With increasing drought stress, the proportion of dark fixation increases, the CAM plant becomes richer in 13 C. The δ- 13 C value of a CAM plant is therefore a good measure of the drought stress at its place of growth, as it provides information about the extent of the CAM metabolism in relation to the normal C 3 metabolism. With a good water supply, optional CAM plants and those with CAM cycling show δ- 13 C values which correspond to those of C 3 plants.
Comparison between C 3 , C 4 and CAM plants
feature | C 3 | C 4 | CAM |
---|---|---|---|
Perspiration quotient [ml (H 2 O) per g (C)] | 450-900 | 250-350 | 18–100 (during the night) or 150–600 (during the day) |
Water use efficiency (dry weight produced in g per g water loss) | 1.05-2.22 | 2.85-4.00 | 8.0-55.0 |
maximum photosynthesis rate [µmol fixed CO 2 / leaf area m 2 · second] | 20-40 | 30-60 | 5–12 (in the light) or 6–10 (in the dark) |
Temperature optimum | 15-25 ° C | 30-47 ° C | 35 ° C |
Gain in dry matter ([tons / hectare · year]) | 10-25 | 40-80 | 6-10 |
δ- 13 C values | −33 to −22 ‰ | −17 to −9 ‰ | −17 to −9 ‰ (drought) or −32 to −20 ‰ (well supplied with H 2 O); constitutive CAM: −18 to −12 ‰ |
evolution
Generally falling CO 2 values in the atmosphere are considered to be the mainspring for the Crassulacean acid metabolism . The earliest possible point in time for the first evolutionary origins is at the transition from the late Carboniferous to the early Permian about 300–250 million years ago. It is possible that CAM first evolved in aquatic plants. The origin of more recent CAM lines is largely considered to be around 35 million years before our time. CAM is a beneficial "answer" to water scarcity; However, evolutionary biologists assume that there were mechanisms for saving water and also for water storage before CAM, for example protection against evaporation and succulence or a thickening of the epidermins or the wax layer (photoprotection), hair (reduction of dehydration). However, these adaptations make CO 2 uptake more difficult (closed stomata, low diffusion of CO 2 in succulent tissues). Therefore, CAM is primarily viewed as a response to low CO 2 levels , but falling carbon dioxide levels are not the sole selection criterion for CAM.
How today's CAM plants developed from their C 3 ancestors is still the subject of research. In C 3 plants there are basic functions for a CAM metabolism in the guard cells of the stoma. It was therefore suggested that these were transferred into the mesophyll cells in the course of evolution towards CAM. Plants that practice CAM cycling are considered to be an important intermediate step towards constitutive CAM. It is therefore believed that one of the first evolutionary steps towards CAM was the ability to re-fix carbon dioxide from breathing during the night. Nowadays, this mainly happens with succulent plants. Presumably, the C have 3 -Vorfahren this succulence already shown. A second important step is the appearance of special translocators in the vacuole. They prevent C 4 acids from diffusing into the cytosol in an uncontrolled manner and thus adversely changing the pH value. The enzymes required for the CAM metabolism are also found in C 3 plants. This has made it possible for CAM plants to emerge in different, unrelated families in monocots and dicotyledons from different habitats. Due to the selection advantages associated with CAM in the case of drought, salinity and especially low CO 2 concentrations, CAM plants (such as C 4 plants) have " developed " several times and independently of one another from C 3 precursor plants in the course of evolution .
use
CAM plants are only used agriculturally to a limited extent. They are mainly cultivated in areas where the cultivation of C 3 and C 4 plants is not worthwhile due to high evapotranspiration and insufficient rainfall . The pineapple, the prickly pear species Opuntia ficus-indica , the sisal agave and the blue agave are CAM plants that have achieved agricultural importance.
Pineapple has its most favorable growing areas in the tropics , especially in South Africa and Australia . Every year and hectare , about 86 tons of fruit are obtained from the cultivation of pineapples . In 2003, the international trade value of the pineapple was $ 1.9 billion.
The prickly pear was mainly used commercially at the beginning of the 20th century. Nowadays it is mainly grown as food and forage in many parts of the world. The annual yield is 47–50 tons per hectare.
Agaves are grown around the world, mostly for fiber or to make alcoholic beverages . This is how the eponymous sisal fibers are obtained from the sisal agave . Production was highest in the 1960s. After that it sank continuously as the sisal fiber was replaced more and more by synthetic fibers made of polypropylene . In 2006, 246,000 tons were produced.
Another important agave species is the blue agave, from which 50 tons of dry matter can be harvested per hectare and year. After the leaves have been chopped off, the sugar syrup obtained from the trunk (the “Cabeza”) is fermented and processed into tequila . The sugar syrup of other types of agave is also distilled, for example into mezcal .
Due to the fact that water consumption is ten times lower than that of C 3 and C 4 plants, CAM plants can also be used for bioenergy use in semi-arid (semi-arid) areas in which hardly any or no food crops are grown and thus only one there is little competition for land for food crops. It is estimated that 4 to 15% of the semi-arid area in question has a bioenergy potential of around 5 petawatt hours , which corresponds to the worldwide annual electricity generation from gas-fired power plants .
The high sugar content of blue agave is already used today for the production of bioethanol . Mexico and large parts of the Karoo in Southeast Africa, in particular, have been taken into closer consideration for cultivation, as these locations are unsuitable for most other crops. The annual production of ethanol from the blue agave is 14,000 liters per hectare, possibly this can be increased to 34,000 liters of ethanol per hectare.
Storage of citrate and isocitrate
In ice herb and other CAM plants of the genus "Clusia", citric acid is stored in the vacuoles in addition to malic acid . Citrate is a metabolic intermediate of the citric acid cycle formed in the mitochondria . During the night, like malate, this is stored in the vacuole with consumption of ATP (there as citric acid). During the day it is decarboxylated to α-ketoglutarate in the cytoplasm by an NADP-dependent isocitrate dehydrogenase (IDH) . Alternatively, citrate is brought directly into the mitochondria and converted there by an NAD-dependent IDH. In both cases, α-ketoglutarate is metabolized to pyruvate in further steps of the citric acid cycle. In the CAM tree Clusia alata this happens after malate has been decarboxylated, which means that both processes run independently of each other.
The biological significance is not fully understood, especially since the nightly storage of citrate, in contrast to that of malate, is not associated with any net gain in carbon, but is somewhat more favorable in terms of energy. A number of functions have been suggested. The decarboxylation of citrate could serve as a protective measure against the photoinhibition that occurs at high levels of exposure . CAM plants in particular are often exposed to very high levels of light. The process can also contribute to the redox equilibrium in the cell. It was also discussed that the stress caused by ROS could be countered. ROS form due to the high and long exposure levels.
Finally, during the CAM idle (see section above ), it was suggested that incorporating citric acid into the vacuoles gave the plant an advantage. Citrate serves as a buffer substance and thus reduces the electrochemical proton gradient on the tonoplast. Thus, citrate allows more malate to be stored during the night.
In addition to citrate, isocitrate can also be stored in the vacuoles.
literature
- Andreas Bresinsky, Christian Körner, Joachim W. Kadereit, G. Neuhaus, Uwe Sonnewald: Strasburger - textbook of botany. 36th edition. Spectrum Akademischer Verlag, Heidelberg 2008, ISBN 978-3-8274-1455-7 .
- Hans W. Heldt , Birgit Piechulla: Plant biochemistry . 4th edition. Spectrum Akademischer Verlag, Heidelberg 2008, ISBN 978-3-8274-1961-3 .
- Caroline Bowsher, Martin W. Steer, Alyson K. Tobin: Plant Biochemistry . Garland Pub, New York, NY 2008, ISBN 978-0-8153-4121-5 .
- Ulrich Lüttge, Manfred Kluge, Gabriela Bauer: Botany. 5th, completely revised edition. Wiley-VCH, Weinheim 2005, ISBN 3-527-31179-3 .
- Ulrich Lüttge: Ecophysiology of Crassulacean Acid Metabolism (CAM). In: Annals of Botany. Volume 93, Number 6, 2004, pp. 629-652. PMID 15150072 . PDF (free full text access)
- Peter H. Raven, Ray F. Evert, Susan E. Eichhorn: Biology of plants. 4th edition. Gruyter, Berlin, New York 2006, ISBN 3-11-018531-8 .
- Katia Silvera, Kurt M. Neubig, W. Mark Whitten, Norris H. Williams, Klaus Winter, John C. Cushman: Evolution along the crassulacean acid metabolism continuum. In: Functional Plant Biology. Volume 37, number 11, 2010, pp. 995-1010, doi: 10.1071 / FP10084 .
Individual evidence
- ^ Neil A. Campbell, Jane B. Reece , Jürgen Markl: Biology. 6th edition. Spektrum Akademischer Verlag, 2003, ISBN 3-8274-1352-4 , p. 227.
- ↑ a b c Gerhard Richter: Metabolic physiology of plants: Physiology and biochemistry of the primary and secondary metabolism. 6., completely reworked. Edition. Thieme, Stuttgart 1998, ISBN 3-13-442006-6 , p. 187.
- ^ T. de Saussure: Recherches chimiques sur la végétation. Chez la V. Nyon, Paris 1804.
- ^ B. Heyne: On the deoxidation of the leaves of Cotyledon calycina. In: Trans Linn Soc Lond. 11, 1815, pp. 213-215.
- ↑ JT Beatty Govindjee, H. Gest: Discoveries in photosynthesis . Springer, 2005, ISBN 1-4020-3323-0 , p. 883.
- ↑ a b A. M. Borland et al .: Orchestration of carbohydrate processing for crassulacean acid metabolism. In: Curr Opin Plant Biol. Volume 31 , 2016, p. 118-124 , doi : 10.1016 / j.pbi.2016.04.001 , PMID 27101569 .
- ↑ a b Hans W. Heldt, Birgit Piechulla: Plant biochemistry . 4th edition. Spektrum Akademischer Verlag, Heidelberg 2008, ISBN 978-3-8274-1961-3 , p. 227.
- ^ A b H. Robert Horton, Laurence A. Moran, K. Gray Scrimgeour, Marc D. Perry, J. David Rawn: Biochemistry. 4th updated edition. Pearson Studium, 2008, ISBN 978-3-8273-7312-0 , p. 637.
- ↑ a b Hans W. Heldt, Birgit Piechulla: Plant biochemistry . 4th edition. Spektrum Akademischer Verlag, Heidelberg 2008, ISBN 978-3-8274-1961-3 , p. 229.
- ↑ Ernst-Detlef Schulze, Erwin Beck, Klaus Muller-Hohenstein: Plant Ecology . Springer, Berlin 2005, ISBN 3-540-20833-X , p. 138.
- ^ Peter Karlson, Detlef Doenecke, Jan Koolman, Georg Fuchs, Wolfgang Gerok: Karlsons Biochemie und Pathobiochemie. 15th edition. Georg Thieme, 2005, ISBN 3-13-357815-4 , p. 423.
- ↑ a b c d Ernst-Detlef Schulze, Erwin Beck, Klaus Muller-Hohenstein: Plant Ecology . Springer, Berlin 2005, ISBN 3-540-20833-X , p. 139.
- ^ Ulrich Lüttge , Manfred Kluge, Gabriela Bauer: Botany. 5th, completely revised. Edition. Wiley-VCH, Weinheim 2005, ISBN 3-527-31179-3 , p. 483.
- ↑ a b c d e Andreas Bresinsky, Christian Körner, Joachim W. Kadereit, Gunther Neuhaus, Uwe Sonnewald: Strasburger - Textbook of Botany. Founded by E. Strasburger. 36th edition. Spektrum Akademischer Verlag, Heidelberg 2008, ISBN 978-3-8274-1455-7 , p. 314.
- ^ IC Buchanan-Bollig, JAC Smith: Circadian rhythms in crassulacean acid metabolism: phase relationships between gas exchange, leaf water relations and malate metabolism in Kalanchoë daigremontiana. In: Planta. 161 (4), 1984, pp. 314-319. doi: 10.1007 / BF00398721
- ^ A. Herrera: Crassulacean acid metabolism and fitness under water deficit stress: if not for carbon gain, what is facultative CAM good for? In: Ann Bot. 103 (4), 2009, pp. 645-653. PMID 18708641 . doi: 10.1093 / aob / mcn145
- ↑ a b K. Winter et al .: On the nature of facultative and constitutive CAM: environmental and developmental control of CAM expression during early growth of Clusia, Kalanchoe, and Opuntia. In: J Exp Bot. 59 (7), 2008, pp. 1829-1840. PMID 18440928 ; PDF (free full text access)
- ↑ a b c d J. C. Cushman: Crassulacean acid metabolism. A plastic photosynthetic adaptation to arid environments. In: Plant Physiol. 127 (4), 2001, pp. 1439-1448. PMID 11743087 ; PDF (free full text access)
- ↑ a b c d Hans Lambers, F. Stuart Chapin, Thijs L. Pons: Plant Physiological Ecology. 2nd Edition. Springer, Berlin 2008, ISBN 978-0-387-78340-6 , p. 80.
- ^ A b c Ernst-Detlef Schulze, Erwin Beck, Klaus Muller-Hohenstein: Plant Ecology. Springer, Berlin 2005, ISBN 3-540-20833-X , p. 137.
- ↑ U. Lüttge: Carbon dioxide and water demand: Crassulacean acid metabolism (CAM), a versatile ecological adaptation exemplifying the need for integration in ecophysiological work. In: New Phytologist. 106 (4), 1987, pp. 593-629. PDF (free full text access)
- ↑ K. Winter et al .: Canopy CO 2 exchange of two neotropical tree species exhibiting constitutive and facultative CAM photosynthesis, Clusia rosea and Clusia cylindrica. In: J Exp Bot. 60 (11), 2009, pp. 3167-3177. PMID 19487388 . PMC 2718218 (free full text)
- ↑ K. Winter, JA Holtum: Environment or development? Lifetime net CO 2 exchange and control of the expression of Crassulacean acid metabolism in Mesembryanthemum crystallinum. In: Plant Physiol. 143 (1), 2007, pp. 98-107. PMID 17056756 . PMC 1761986 (free full text)
- ↑ a b c d e U. Lüttge: Ecophysiology of Crassulacean Acid Metabolism (CAM). In: Annals of Botany. 93 (6), 2004, pp. 629-652. PMID 15150072 ; PDF (free full text access)
- ↑ HG Nimmo: How to tell the time: the regulation of phosphoenolpyruvate carboxylase in Crassulacean acid metabolism (CAM) plants. In: Biochem Soc Trans . 31 (Pt 3), 2003, pp. 728-730. PMID 12773193 . PDF (free full text access)
- ↑ K. Silvera et al .: Multiple isoforms of phosphoenolpyruvate carboxylase in the Orchidaceae (subtribe Oncidiinae): implications for the evolution of crassulacean acid metabolism . In: J Exp Bot. Volume 65 , no. 13 , 2014, p. 3623-3636 ., Doi : 10.1093 / jxb / eru234 , PMID 24913627 .
- ↑ a b c d K. Heyduk et al .: Evolution of a CAM anatomy predates the origins of Crassulacean acid metabolism in the Agavoideae (Asparagaceae) . In: Mol Phylogenet Evol. tape 105 , 2016, p. 102-113 , doi : 10.1016 / j.ympev.2016.08.018 , PMID 27591171 .
- ↑ Caroline Bowsher, Martin W. Steer, Alyson K. Tobin: Plant Biochemistry . Garland Pub, New York, NY 2008, ISBN 978-0-8153-4121-5 , p. 134.
- ↑ a b c d U. Lüttge: Ecophysiology of CAM photosynthesis. In: Jaume Flexas, Francesco Loreto, Hipólito Medrano (Eds.): Terrestrial Photosynthesis in a Changing Environment: A Molecular, Physiological, and Ecological Approach . Cambridge University Press, 2012, ISBN 978-0-521-89941-3 , pp. 71-84.
- ↑ JA Holtum et al .: Australia lacks stem succulents but is it depauperate in plants with crassulacean acid metabolism (CAM)? In: Curr Opin Plant Biol. 31, 2016, pp. 109–117. doi: 10.1016 / j.pbi.2016.03.018 . PMID 27088716
- ^ AN Dodd et al .: Crassulacean acid metabolism: plastic, fantastic. In: J Exp Bot. 53 (369), 2002, pp. 569-580. PMID 11886877 ; PDF (free full text access)
- ↑ a b c Ulrich Lüttge, Manfred Kluge, Gabriela Bauer: Botany. 5th, completely revised. Edition. Wiley-VCH, Weinheim 2005, ISBN 3-527-31179-3 , p. 485.
- ↑ Hans W. Heldt, Birgit Piechulla: Plant biochemistry . 4th edition. Spektrum Akademischer Verlag, Heidelberg 2008, ISBN 978-3-8274-1961-3 , p. 226.
- ^ Ulrich Lüttge, Manfred Kluge, Gabriela Bauer: Botany. 5th, completely revised. Edition. Wiley-VCH, Weinheim 2005, ISBN 3-527-31179-3 , p. 484.
- ↑ a b U. Lüttge: CO 2 -concentrating: consequences in crassulacean acid metabolism. In: J Exp Bot. 53 (378), 2002, pp. 2131-2142. PMID 12379779 ; PDF (free full text access)
- ↑ Caroline Bowsher, Martin W. Steer, Alyson K. Tobin: Plant Biochemistry . Garland Pub, New York, NY 2008, ISBN 978-0-8153-4121-5 , p. 136.
- ↑ a b c d R. E. Bone et al .: A macro-ecological perspective on crassulacean acid metabolism (CAM) photosynthesis evolution in Afro-Madagascan drylands: Eulophiinae orchids as a case study. In: New Phytologist . tape 208 , no. 2 , 2015, p. 469-481 , doi : 10.1111 / nph.13572 , PMID 26192467 .
- ↑ JE Keeley, RK Monson, PW Rundel: Evolution of photosynthesis II: evolution and expansion of CAM and C4 photosynthetic types. In: J. Flexas, F. Loreto, H. Medrano (Eds.): Terrestrial photosynthesis in a changing environment: a molecular, physiological and ecological approach. Cambridge University Press, Cambridge, UK 2012, pp. 386-397.
- ^ A b Caroline Bowsher, Martin W. Steer, Alyson K. Tobin: Plant Biochemistry . Garland Pub, New York, NY 2008, ISBN 978-0-8153-4121-5 , pp. 133f.
- ^ Mary J. West-Eberhard: Developmental Plasticity And Evolution . Oxford University Press 2003, ISBN 0-19-512235-6 , p. 512.
- ↑ a b A. M. Borland et al .: Exploiting the potential of plants with crassulacean acid metabolism for bioenergy production on marginal lands. In: J Exp Bot. 60 (10), 2009, pp. 2879-2896. PMID 19395392 ; PDF (free full text access)
- ↑ Thomas Heller: Agaves. Natur und Tier-Verlag, Stuttgart 2003, ISBN 3-931587-89-4 , p. 33.
- ↑ PM Mason et al .: The potential of CAM crops as a globally significant bioenergy resource: moving from 'fuel or food' to 'fuel and more food'. In: Energy and Environmental Science . 8, 2015, pp. 2320–2329, doi: 10.1039 / c5ee00242g
- ↑ Ulrich Lüttge, Manfred Kluge, Gerhard Thiel: Botany - The comprehensive biology of plants. Wiley-VCH Verlag, 2010, ISBN 978-3-527-32030-1 , pp. 792f.
- ↑ a b A. Kornas et al .: Adaptation of the obligate CAM plant Clusia alata to light stress: Metabolic responses. In: J Plant Physiol. 166 (17), 2008, pp. 1914-1922. PMID 19592134 . doi: 10.1016 / j.jplph.2009.06.005
- ^ L. Freschi et al .: Correlation between citric acid and nitrate metabolisms during CAM cycle in the atmospheric bromeliad Tillandsia pohliana. In: J Plant Physiol. 167 (18), 2010, pp. 1577-1583. PMID 20594612 . doi: 10.1016 / j.jplph.2010.06.002
- ↑ Ulrich Lüttge, Manfred Kluge, Gerhard Thiel: Botany - The comprehensive biology of plants . Wiley-VCH Verlag, 2010, ISBN 978-3-527-32030-1 , p. 796.