C 4 plant
Name-giving C 4 molecules with a blue chain of four carbon atoms |
L-aspartic acid |
L-malic acid |
C 4 plants use a metabolic pathway to first pre-fix carbon dioxide for photosynthesis and only then, like C 3 plants, build up into carbohydrates in the Calvin cycle (C 4 photosynthesis). The name C 4 is derived from the first fixation product, which is created through the assimilation of carbon dioxide. While in C 3 plants this is a carbon compound with three C atoms ( D -3 phosphoglycerate ), the first thing to be found in C 4 plants is oxaloacetate , a compound with four C atoms.
The carbon dioxide assimilation and the Calvin cycle take place spatially separated from each other in C 4 plants. By applying energy, carbon dioxide is actively enriched, which leads to a higher rate of photosynthesis - especially when there is a lack of water and the resulting narrowing of the stomata . Therefore, C 4 plants are ecophysiologically superior to C 3 plants under arid conditions. Due to the active enrichment, the photorespiration takes place significantly less often. Typical C 4 plants are in particular grasses , including well-known useful plants such as maize , sugar cane and millet , but also other species such as amaranth .
Plants with a Crassulacean acid metabolism proceed in a similar way to C 4 plants, but with them the pre-fixation and the Calvin cycle are separated in time.
Occurrence of C 4 photosynthesis in the plant kingdom

About 3% of Bedecktsamer operate C 4 -Photosynthese. Most (approx. 80%) belong to the grasses , especially the sweet grasses , followed by sedges . The majority of the fast-growing agricultural plants are C 4 plants, but many of the weeds classified as very stubborn (e.g. barnyard millet , blood-red foxglove or tuberous sedge ) also fall under this category . But this metabolic path is also found in a number of dicotyledons , especially in the foxtail family and other carnation-like plants , in milkweed plants and occasionally in bindweed plants and daisy plants . In the foxtail family (Amaranthaceae) there are both C 3 and C 4 species in the report genus . Well-known C 4 plants are amaranth , millet , maize , sugar cane and giant Chinese reed .
Since the C 4 photosynthesis arose at least 65 times independently of one another in 19 different families (as of 2017), one speaks of a polyphyletic characteristic.
Compared to C 3 plants, C 4 plants stand out when there is a shortage of water, high temperatures and solar radiation. Therefore, they have an advantage over other plant species in these same climatic zones. About 70% of all species living in Death Valley National Park operate a C 4 photosynthesis. In general, the majority of all C 4 grasses grow in regions with a geographical latitude of less than 30 degrees . They are less common in cold regions, for example in the boreal zone between the 50th and 65th parallel and at high altitudes. The treeless tundra of the alpine zone, where it is dry, is an exception . The C 4 grass species Orinus thoroldii was also discovered in Tibet at an altitude of around 5200 meters . Generally, they do not occur in polar and sub-polar regions (beyond the 65th parallel).
There are some cold-tolerant C 4 plants that can withstand frost and winter temperatures (−20 ° C), for example C 4 grasses in the Andes . C 4 plants also grow in cool regions such as the coast of New Zealand , the Atlantic coasts of Canada and Great Britain or some swamp areas . No C 4 plants were identified in the case of naked samers , mosses or cryptogams . It is not yet known why - with a few exceptions - there are no trees with a C 4 photosynthesis. Only a few rare species have been discovered in Hawaii , for example Euphorbia olowaluana or E. Herbstii .
Although C 4 plants only make up around 5% of biomass and 3% of plant species worldwide , they are responsible for 23% of the fixation of carbon dioxide. In the last thirty years, a spread of C 4 plants has also been observed in warm, sunny locations in Central Europe. Mostly it is millet-like grasses and foxtail species. At least so far, their spread has not been seen as a threat to native flora.
identification
In order to be able to differentiate between C 3 and C 4 plants, various methods are used: The leaf anatomy (see section below ) already provides initial clues. In addition, the primary photosynthetic product can be identified by radioactive isotopes ( 14 C) (short-term 14 CO 2 fixation). The CO 2 compensation point also allows conclusions to be drawn about the metabolic type. C 4 plants are characterized by very little or no measurable photorespiration . Finally, from the 13 C / 12 C isotope ratio in the carbon of the plant, a C 3 - C 4 photosynthesis can be inferred (see section below ).
discovery
Regardless of the importance of the underlying metabolic pathway, morphological and plant anatomical peculiarities of C 4 plants were observed at the end of the 19th century and the beginning of the 20th century. This is how Gottlieb Haberlandt identified the crown anatomy (see section below) in various grasses. The formation and accumulation of starch that takes place in the bundle sheath cells was described in the literature in 1944. In 1955, differently pronounced chloroplasts in the bundle sheath cells or mesophyll cells (dimorphological / dimorphogenic chloroplasts) provided an indication of an actually different specialization in the metabolic pathway.
The first biochemical studies on a C 4 plant were carried out by Hugo Kortschak . In the early 1950s he identified L - malate and L - aspartate as the first CO 2 fixation product at a sugar cane research institute in Hawaii by radioactive labeling using 14 CO 2 . These are C 4 compounds and thus contradicted the findings of Melvin Calvin , Andrew Benson and James Bassham . They had shown that the first metabolic product in the CO 2 fixation in the dark reaction is a C 3 compound, 3-phosphoglycerate . The results of Kortschak were initially published unreferenced in the institute's annual report in 1954 and were only mentioned in the scientific literature in 1957 and 1962 after further investigations. However, further clarification was not pursued because the research group did not have the necessary biochemical-enzymological skills and knowledge for further work. On the other hand, Kortschak's results contradicted the generally accepted dogma of (C 3 -) photosynthesis through the work of Melvin Calvin, so that Kortschak was intimidated even after contact with the research group around Calvin. It was not until 1965 that the results were published in a more publicly accessible specialist magazine.
Independently of Kortschak, the Russian Yuri Karpilov discovered in 1960 that the first fixation product was a C 4 compound in maize , but this only became known in the late 1960s. The Australian plant researcher Barry Osmond also came to the conclusion that the first fixative product was malate , through labeling experiments on plants of the genus Report (in particular Atriplex spongiosa ). Due to doubts from the professional world, these results were not published until 1967.
Only the Australian researchers Marshall Davidson Hatch and Charles Roger Slack were able to decode the biochemistry of the metabolic pathway with these results and their own investigations . First, in 1966, a first model was proposed in which a C 3 compound (pyruvate or phosphoenolpyruvate) was carboxylated to a C 4 dicarboxylic acid. The fourth carbon atom of this newly formed dicarboxylic acid is then transferred to an acceptor so that 3-phosphoglycerate is formed from it. The original C 3 compound is regenerated again. In the following years, further knowledge was gained, for example the primary carboxylating enzyme (PEP carboxylase) and other key inter- and intracellular enzymes of the new metabolic pathway were identified. With this data, the researchers were able to draw up a detailed scheme in 1971. As a result, the C 4 metabolism is also known as the hatch -slack path or hatch-slack cycle after its discoverers . Hatch and Slack, on the other hand, referred to the metabolic pathway as the C 4 -dicarboxylic acid pathway .
anatomy

1. CO 2 is pre-fixed (A).
2. The C 4 compound formed from this is transported into the adjacent compartment (B)
3. There, CO 2 is released and consumed in the Calvin cycle.
4. The remaining C 3 body is transported back and regenerated for another cycle.
C 4 plants can often be identified on the basis of a characteristic anatomy of the leaf. Here the vascular bundles are embedded in a first ring of the bundle sheath cells in a ring shape. The bundle sheath cells are then in turn surrounded by the mesophyll cells in a second ring (see figure). Due to the numerous vascular bundles, mesophyll and bundle sheath are present in a ratio of approximately 1: 1. This special structure is also referred to as “wreath anatomy” or after the term “wreath type” coined by Gottlieb Haberlandt in 1896. The SCARECROW root gene plays an important role in developing the crown anatomy of C 4 plants , as shown by experiments on maize plants. The mesophyll surrounding the bundle sheaths is not differentiated into a C 3 -type sponge or palisade parenchyma .
This special arrangement also explains the principle of the C 4 metabolic pathway, which usually extends over two neighboring cell types that have a high degree of functionalization. Here, CO 2 is first pre-fixed in mesophyll cells in a C 4 compound. Mesophyll cells do not contain any RuBisCO. The CO 2 bound in the form of that C 4 compound is transported into bundle sheath cells via particularly numerous plasmodesmata and released there. Due to their enzyme equipment, these can carry out the Calvin cycle . The cell walls of the bundle sheath cells are often suberinized , which means that the released CO 2 can hardly diffuse out of the cell . It accumulates in the cell, so that there is a high CO 2 concentration (about 10 times higher than in the outside air) for the RuBisCO , which it introduces into the Calvin cycle for assimilation . The C 3 compound formed during the release is transported back into the mesophyll cell. The CO 2 pre-fixation (in mesophyll cells) and the actual carbon assimilation in the Calvin cycle (in bundle sheath cells) are thus spatially separated from one another and thus take place in different reaction spaces.
Due to this separation of functions, the chloroplasts of the mesophyll and bundle sheath cells of some C 4 plants also differ . The chloroplasts of the bundle sheath cells receive a lot of strength and the grana are missing. One speaks of a chloroplast dimorphism or dimorphogenic chloroplasts . However, this is not absolutely necessary for the C 4 photosynthesis to proceed.
biochemistry
Differentiation from C 3 and CAM plants
All green plants photosynthesize to build up carbohydrates . In the "dark reaction", carbon dioxide (CO 2 ) is fixed and built up into carbohydrates. Most plants (C 3 plants) operate a mechanism described as C 3 metabolism, in which carbon dioxide passively passes through the stomata into the cells and is fixed as a substrate during the day in the Calvin cycle. This passive diffusion of carbon dioxide into the cells has a disadvantage at high ambient temperatures. Under these conditions, plants have to close their stomata in order to keep water loss through transpiration within limits and not to dry out. Closing the stomata also makes gas exchange and thus the absorption of CO 2 for photosynthesis more difficult. An additional problem is that the enzyme RuBisCo (which carries out the CO 2 fixation in the Calvin cycle) has an oxygenase function in addition to its carboxylase function . A low CO 2 partial pressure, a high O 2 partial pressure and high temperatures favor the oxygenase function of the RuBisCO. Ribulose-1,5-bisphosphate is consumed during the undesired oxidation and has to be regenerated in a complex process using so-called photorespiration .
C 4 plants counter the problem of providing carbon dioxide through a special mechanism. The CO 2 concentration for the fixation is increased actively and thus with energy consumption . There is a spatial separation (two cell types: mesophyll cells and vascular bundle sheath cells) for the pre-fixation and metabolization of carbon dioxide. This allows the plants to partially close their stomata because, unlike C 3 plants, they are not restricted by the simple diffusion of carbon dioxide into the cells. The downstream CO 2 fixation in the Calvin cycle, however, corresponds to that of C 3 plants (“dark reaction”). The division of labor between the two reaction chambers requires that both tissues have a different set of enzymes involved.
The pre-fixing enzyme PEP carboxylase has a much higher affinity for CO 2 than RuBisCO, which only carries out the secondary fixation here. Since the first tangible fixation product is a C 4 body, oxaloacetate , this name was chosen to distinguish it from plants with a “normal” C 3 photosynthetic metabolism.
The prefixing of the C 4 plants is similar to that of the CAM plants . However, the latter is a temporal separation of pre-fixation and Calvin cycle, while C 4 plants perform a spatial separation.
Biochemistry in the mesophyll cell
The C 4 metabolism works in principle as an upstream CO 2 pump, which begins with the fixation of CO 2 in the form of bicarbonate (HCO 3 - ) in the mesophyll cell. The formation of HCO 3 - from CO 2 is catalyzed by a carbonic anhydrase and takes place in the cytosol.
A phosphoenolpyruvate carboxylase (PEPC) catalyzes the irreversible condensation of a molecule of phosphoenolpyruvate (PEP) with HCO 3 - , so that oxaloacetate is formed in addition to phosphate . PEPC has a very high affinity for PEP. Oxaloacetate is converted into L - malate (" malate former"), in some C 4 plants also into L - aspartate . The carbon dioxide is in equilibrium with the CO 2 of the intracellular space and thus ultimately with the outside air. Since it is already withdrawn from equilibrium in the bundle sheath cell, this facilitates post-diffusion. In addition, PEPC is not inhibited by oxygen.
![]() |
HCO 3 - P i PEP-C![]() |
![]() |
Phosphoenolpyruvate (PEP) | Oxaloacetate |
Pyruvate transported back into the mesophyll cell enters the chloroplast through a specific translocator, with protons or sodium ions being transported with it. There a pyruvate phosphate dikinase converts pyruvate into PEP. In this unusual reaction, both ATP and inorganic phosphate are consumed. In fact, the middle phosphate from ATP (P β ) is incorporated into pyruvate.
The pyrophosphate (PP i ) released during the reaction is hydrolyzed by a pyrophosphatase and thus thermodynamically withdrawn from equilibrium. This catalysis is therefore irreversible. In turn, PEP enters the cytosol through a specific antiporter in exchange for inorganic phosphate (PEP-phosphate translocator) and can thus condense with bicarbonate again.
Reactions in the bundle sheath cell
How the carbon dioxide is released again and fed into the Calvin cycle is different for C 4 plants. So far, three basic types of C 4 photosynthesis have been identified, which differ in the provision of CO 2 during decarboxylation in the bundle sheath cell:
- NADP + malate enzyme type ( NADP-ME ): Malate is oxidatively decarboxylated in the chloroplasts, which catalyzes an NADP + -dependent malate enzyme , thereby releasing NADPH . Typical representatives are maize, sugar cane or carrot millet .
- NAD + malate enzyme type ( NAD-ME ): Here malate is decarboxylated in the mitochondria , which catalyzes an NAD + -dependent malate enzyme with consumption of NAD + . The released carbon dioxide diffuses into the chloroplasts. Curved amaranth , millet or purslane operated this type of CO 2 release.
- PEP carboxykinase type ( PEPCK ): Two cycles run in parallel here. Once, as in the NAD type, malate is converted into the mitochondria. Oxaloacetate is mainly decarboxylated in the cytosol by a PEP carboxykinase, which gives it its name, using ATP . This C 4 -Stoffwechseltyp found in fast-growing tropical grasses such as in Guinea Grass ( Megathyrsus maximus ) or Chloris gayana .
While all three types have many enzyme reactions in the mesophyll cell in common (for example the way to oxaloacetate and the regeneration of PEP in the chloroplasts), they differ in the release of CO 2 in the bundle sheath cell.
After the CO 2 has been released , phosphoenolpyruvate (PEP) or pyruvate are transported back into the mesophyll cell by plasmodesmata. In plants with the NAD malate enzyme type, pyruvate is first converted to L - alanine by transamination and this is then transported.
A diffusion-induced backflow of carbon dioxide is contained by the suberine layer. Should CO 2 get back into the mesophyll cell, it will be captured again by the carbonic anhydrase activity.
The bidirectional material transport takes place via diffusion. This assumes that a sufficiently high concentration gradient between the two cells is created and maintained. Since the transition metabolites (e.g. malate, pyruvate) are quickly consumed at the place of their determination, this concentration gradient can be achieved.
NADP malate enzyme type (NADP-ME)
The NADP malate enzyme type is the simplest of all three types. After oxaloacetate has been formed in the cytosol of the mesophyll cell, it is brought into the chloroplasts by a specific translocator (oxaloacetate / malate antiporter). There, an NADP + -dependent malate dehydrogenase ( EC 1.1.1.82 ) converts oxaloacetate into L- malate while consuming NADP . After it has got out of the chloroplasts again, it passes through the plasmodesmata to the bundle sheath cells and is transported into the chloroplasts. There, the eponymous NADP + -dependent malate enzyme decarboxylates malate to pyruvate, whereby NADP + is reduced. Pyruvate leaves the chloroplast via a specific translocator and returns to the mesophyll cell through the plasmodesmata. In the chloroplasts this is converted to PEP as described above and the cycle is closed.
Plants with the NADP-dependent malate enzyme often contain relatively large chloroplasts and hardly any mitochondria in their bundle sheath cells . In addition, the amount of grana stacks varies from nonexistent (e.g. in sugar cane and millet) to half as many compared to the mesophyll cell (foxtail family); Stroma lamellas are available. Accordingly, the photosystem II (PS II), which is normally located in the grana membranes of the thylakoids , is hardly active in them . On the one hand, this means that hardly any oxygen is produced by photosynthesis, which further lowers the risk of photorespiration. On the other hand, there is no possibility of linear electron transport in the light reaction of photosynthesis, which normally generates the NADPH required in the Calvin cycle. With only one active photosystem ( photosystem I ), cyclic photophosphorylation is primarily operated, so that ATP is generated. NADPH must therefore be made available from the mesophyll cell by the light reaction of photosynthesis. However, it is not transported directly into the bundle sheath cell. Instead, it is indirectly transferred (together with ATP) via a triose phosphate-3-phosphoglycerate shuttle into the chloroplasts of the bundle sheath cells. Dihydroxyacetone phosphate is usually used here . Alternatively, NADPH is formed in the course of the C 4 metabolism, when malate is decarboxylated to pyruvate in the bundle sheath cell.
NAD malate enzyme type (NAD-ME)
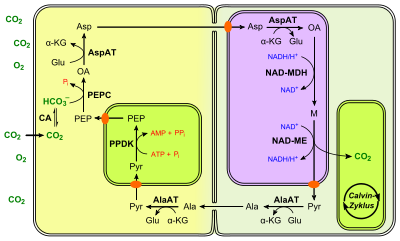
This type is a little more complicated than the NADP-ME way. In the mesophyll cells, a cytosolic aspartate aminotransferase converts oxaloacetate into L- aspartate. The amino group of L -glutamate is used for this, so that it becomes α-ketoglutarate. Instead of malate, aspartate is used as a vehicle for carbon transport. The aspartate formed reaches the bundle sheath cell via the plasmodesmata and is transported there into the mitochondria. There it is first converted back to oxaloacetate by a mitochondrial isoenzyme , with α-ketoglutarate at the same time reacting to L -glutamate. Oxaloacetate is then reduced to malate by a mitochondrial NAD + -dependent malate dehydrogenase with consumption of NADH. Finally, the release of CO 2 takes place through the eponymous NAD + -dependent malate enzyme ( EC 1.1.1.39 ) and diffuses the chloroplasts in close proximity. The NADH obtained in this reaction is then available again for the oxidation of oxaloacetate. In contrast to the NADP-ME type, the pyruvate produced is not brought back into the mesophyll cell directly via plasmodesmata, but as L -alanine. This is mutually converted in both cells by an alanine aminotransferase, with L- glutamate also acting as an amino donor. Pyruvate is finally regenerated to PEP as previously described. The different concentration gradients result in a directed diffusion into the mesophyll cell.
In contrast to the NADP type, the chloroplasts of the bundle sheath cells show a high PS II activity. In addition, there are many more and more often larger mitochondria included.
PEP carboxykinase type (PEPCK)
The PEPCK path is the most complex of all three paths, as two cycles run in parallel here.
The one much more strongly operated cycle is based on a transport of L -aspartate. This is created by a cytosolic aspartate aminotransferase from oxaloacetate, similar to the NAD-ME type. L- aspartate is transported via the plasmodesmata into the bundle sheath cell, where it is first converted to oxaloacetate (also by a cytosolic aspartate aminotransferase). The name on which this subtype is based is based on a reaction in which oxaloacetate is decarboxylated to PEP. This is namely catalyzed by a PEP carboxykinase ( EC 4.1.1.49 ) with ATP consumption. PEP diffuses back into the mesophyll cell without further reaction and closes the cycle there.
The second operated route is similar to the NADP-ME route. Cytosolically produced oxaloacetate is converted into L- malate in the chloroplasts of the mesophyll cell , which catalyzes an NADP + -dependent malate dehydrogenase with NADPH consumption. This reaches the bundle sheath cell via the plasmodesmata, but is transported into the mitochondria in contrast to the NADP-ME pathway. There an NAD + -dependent malate enzyme releases carbon dioxide (analogous to the NAD-ME pathway). In the PEPCK type, the ATP requirement of the bundle sheath cells is increased by the PEPCK reaction. To cover this, the mitochondria use the released NADH for the respiratory chain for ATP synthesis. Pyruvate brought from the mitochondria does not pass directly through the plasmodesmata back into the mesophyll cell, but via alanine. As with the NAD-ME pathway, this is converted via mutual conversion by an alanine aminotransferase.
The grana in the chloroplasts of the mesophyll and bundle sheath cells are somewhat equally pronounced.
regulation
Three key enzymes necessary for the division of labor are regulated by light, so that the underlying C 4 metabolism is only carried out during the day.
- PEP carboxylase (PEPC) is in an inactive form during the night. The affinity is greatly reduced compared to its substrate PEP. In addition, PEPC is effectively inhibited by malate. This is to prevent PEP from being unnecessarily irreversibly consumed. However, the enzyme activity is - analogously to CAM plants - also regulated by reversible phosphorylation on a serine residue . During the day, a protein serine kinase is activated, which in turn transfers phosphate groups to PEPC. As a result, PEPC is converted into its catalytically active form on exposure to light. The active, phosphorylated form is also much less sensitive to malate. In the dark, a responsible phosphatase dephosphorylates PEPC and thus inactivates it.

- Pyruvate phosphate dikinase (PPDK) is also regulated by light phosphorylation. This is done by a bifunctional serine / threonine kinase - the regulator protein (PDRP or RP for short). It catalyzes both the transfer of a phosphate group to a threonine residue of the PPDK (kinase function) and its removal (phosphatase function). Interestingly, in contrast to most kinases, the regulator protein does not use ATP, but ADP as a P donor. In addition, unlike most phosphatases, dephosphorylation does not take place by means of water, but by inorganic phosphate. In contrast to PEPC, the phosphorylated form of PPDK is inactive. ADP, the substrate for the kinase, is also a strong, competitive inhibitor of phosphatase. Therefore, PDRP is regulated by the ADP level of the stroma: the ADP concentration is low under light, but high in the dark. As a result, PPDK is dephosphorylated under light and thus switched on. In the dark it happens the other way around, PDRP phosphorylates PPDK, which inactivates it.
- Another control mechanism is found with NADP malate dehydrogenase. Similar to other chloroplastic enzymes (e.g. ribulose phosphate kinase or fructose-1,6-bisphosphatase ), this is regulated by the redox state in a light-dependent manner. Reduced thioredoxin mediates their activation by reducing a disulfide bridge (–S – S–) on the outside of each protein to cysteine (–SH). That thioredoxin itself is formed by ferredoxin , which catalyzes a ferredoxin thioredoxin reductase . Ferredoxins are only produced in photosystem I under exposure.
Special forms of C 4 photosynthesis
C 4 photosynthesis without a coronal anatomy
Land plants have been discovered which, although they carry out a C 4 photosynthesis, do not have a coronal anatomy like most C 4 plants. Four species belonging to the goosefoot family, Bienertia sinuspersici , Bienertia cycloptera , Suaeda aralocaspica and Bienertia kavirense , carry out this metabolic pathway within a cell.
These plants grow in deserts areas: Bienertia sinuspersici in countries around the Persian Gulf , Bienertia cycloptera in the region Turkey, Afghanistan and Iran, Bienertia kavirense in the Iranian salt desert ( Dasht-e Kavir ) and Suaeda aralocaspica , a salt plant in Central Asia.
As with C 4 plants with a crown anatomy, C 4 photosynthesis is achieved through a spatial division of CO 2 pre-fixation and actual final fixation in the Calvin cycle, with this taking place intracellularly . The above-mentioned plants have C 4 photosynthesis of the NAD-ME type.
In Suaeda aralocaspica one finds long palisade parenchyma cells , in which the outward (distal) area is used for CO 2 pre-fixation and in which the inward (proximal) area is used for fixation in the Calvin cycle. A large vacuole separates the two reaction spaces. The Bienertia species show a different structure. There is a thin cytosolic compartment on the edge and an unusual central compartment with lots of chloroplasts in the middle. Here, too, there is a spatial division similar to that of the crown anatomy between pre-fixation by PEP and fixation by RuBisCO.
In the C 4 photosynthesis that takes place within a cell, there are two different types of chloroplasts ("dimorphogenic chloroplasts"), which differ in their biochemistry, the storage of starch and in their ultrastructure. The grana are poorly developed in the chloroplasts that lie on the outside , they hardly store any starch and the enzyme PPDK is active there to build up PEP. The other “inward” chloroplasts have RuBisCO, well-developed Grana and store starch. These differences are analogous to those of the respective chloroplasts in mesophyll cells and bundle sheath cells. The cytoskeleton ensures the separation of these functionally different chloroplasts within the cell.
Mitochondria and peroxisomes are also associated with the “inner” chloroplasts . In this way, during the decarboxylation of malate, the carbon dioxide released is brought into close proximity to RuBisCO. In addition, there is an increased probability that the carbon dioxide released during the photorespiratory metabolic pathway will be immediately re-fixed.
Presumably, the marine macroalga Udotea flabellum and the unicellular diatom Thalassiosira weissflogii also carry out C 4 photosynthesis within the same cell.
Facultative C 4 photosynthesis
C 4 photosynthesis has been identified in the ground nettle , a freshwater plant, although it does not have a coronal anatomy. If the CO 2 content of the water is high, C 3 photosynthesis takes place. The ground nettle switches to a C 4 metabolism when the CO 2 concentration drops, so that one speaks of an optional C 4 plant.
Although HCO 3 - is present in higher concentrations in water than CO 2 , the plant absorbs carbon dioxide. This is because their adaxial walls have a low pH value , which shifts the equilibrium of HCO 3 - and CO 2 in favor of CO 2 . CO 2 is pre- fixed and brought into the chloroplast in a C 4 metabolism of the NADP-ME type and enriched there.
At high temperatures, the CO 2 -concentrating C 4 metabolism of the ground nettle has advantages over other C 3 freshwater plants. During the day, the O 2 content of the water increases while the CO 2 content decreases. In addition, CO 2 cannot diffuse into water as quickly as it does in the air , and losses through photorespiration increase. The ground nettle is still able to carry out efficient photosynthesis.
Another representative is, for example, the umbrella rush ( Eleocharis vivipara ), an aquatic plant belonging to the marsh rushes . In the submerged state, it carries out a C 3 photosynthesis. But it switches to C 4 as soon as it grows in the country. Individual stalks above the water surface can also switch to C 4 photosynthesis.
C 4 CAM intermediaries

The Crassulacean acid metabolism (CAM) as the C 4 -Photosynthese a CO 2 -Anreicherungspumpe. The pre- and final fixation of CO 2, however, does not take place spatially as in the case of C 4 plants, but the two processes are separated from one another in time. At the same time, CAM plants also carry out a classic C 3 photosynthesis. This happens, for example, in phase II and IV of obligatory CAM plants. Alternatively, C 3 -CAM species were discovered which only switch to CAM mode after an induced key stimulus such as dryness or salinity. In all cases the same plant cell operates both CAM and a C 3 metabolism.
For C 4 plants, by contrast, there are very few examples that CAM and C 4 metabolism are carried out within the same plant. The majority of C 4 plants are grasses in which no CAM occurs, while C 4 photosynthesis has never been detected in typical CAM plants such as orchids or bromeliads . So you only have in the family of portulacaceae discovered a few species that can use both pathways, such as Portulaca grandiflora , summer purslane or portulaca Mundula . CAM takes place in the succulent cells inside the stem and leaf, while C 4 photosynthesis dominates in the outward leaf cells. Therefore, both metabolic pathways are not operated within the same cell, which indicates that CAM and C 4 photosynthesis are incompatible.
Several reasons are given for this. It is biochemically difficult to regulate both metabolic pathways precisely because of their similarity. In addition, the two routes are based on different anatomical characteristics and transport mechanisms. These specializations are essential for the function, but cannot be combined. Finally, two simultaneous CO 2 concentration measures within a leaf do not result in any ecological advantage. In addition, the first evolutionary steps in development from C 3 plants are fundamentally different.
"C 3 -C 4 intermediate plants"
A number of C 3 plants share basic properties of a C 4 plant. They have a C 4 -type anatomical organization of the leaves with green mesophyll and bundle sheaths and actually concentrate carbon dioxide in the bundle sheaths. In addition, their CO 2 compensation point is between C 3 and C 4 plants. On the other hand, they do not carry out a characteristic transport of C 4 dicarboxylic acids like all C 4 plants.
Such plants were erroneously referred to as “C 3 -C 4 (intermediate) plants” or C 3 -C 4 mixed forms due to their anatomical similarity , but this is not appropriate due to the biochemical differences and the fundamentally different nature of the CO 2 concentration mechanism .
The biochemical basis of these plants is the so-called C 2 photosynthesis , which is based on photorespiratory processes. If RuBisCO uses oxygen instead of carbon dioxide as a substrate, 2-phosphoglycerate is produced , which is regenerated in a complex process. In the course of photorespiration, u. a. two molecules of glycine converted to serine and CO 2 by the glycine decarboxylase complex (GDC). In plants with C 2 (and C 4 ) photosynthesis, GDC is only localized and active in the bundle sheath cells, so that the glycine transported from the mesophyll cells is decarboxylated there and enriched by the chloroplasts. Through this shuttle transport of glycine, a C 2 compound, this special form of metabolism has been called "C 2 photosynthesis". The advantage of this C 2 shuttle is the fact that - if CO 2 were to be released - it would have a very long diffusion path through several cells to the outside of the leaf. This greatly increases the chance of being recaptured. In addition, CO 2 is locally concentrated in the bundle sheath cell due to the GDC activity, so that the photorespiration there is significantly reduced.
C 2 plants have been discovered, for example, in the genera of the salt herbs , solstices , millet , Flaveria and Moricandia .
According to the more recent definition, C 3 -C 4 -intermediate plants are not only considered to be plants that carry out a C 2 -photosynthesis. It also lists plants that are phylogenetically generally between pure C 3 and C 4 plants.
meaning
Ecophysiological aspects
The evolution of the C 4 metabolism is a biochemical adaptation to the falling CO 2 concentration in the atmosphere. Due to the C 4 metabolism with its active CO 2 pump, the plant enjoys several ecological advantages over C 3 plants, since the carbon dioxide concentration around RuBisCO is greatly increased while consuming energy. On the one hand, this considerably reduces photorespiratory losses. While C 3 plants lose at least 30% of the photosynthetically obtained carbon dioxide, C 4 plants can avoid photorespiration even under rising temperatures. This ecophysiological advantage is particularly evident at temperatures above 25 ° C, so that C 4 plants are widely used in hot climates. When the temperature rises, oxygen dissolves better than CO 2 , so that in C 3 plants there are greater losses through photorespiration due to the oxygenase activity of the RuBisCO, which in C 4 plants can be reduced or completely suppressed. It is in these areas that the negative effects of photorespiration from C 3 plants begin to have the greatest effect. Even within a year, dry and hot summers cause a change from C 3 to C 4 grasses on lawns, for example: In the cool spring the meadow bluegrass or the red ostrich grass dominates , but when the summer temperatures are high, C 4 grasses such as the blood red dominate Finger millet become overgrown.
In addition, the C favors 4 -Metabolism under natural growth conditions the nitrogen requirements . It is lower for C 4 plants because they require less RuBisCO. This can work more efficiently due to the higher CO 2 saturation (70 µmol / liter), losses due to photorespiration are minimal. The RuBisCO of the C 3 plants, on the other hand, has to make do with around 10 times lower CO 2 concentrations, so that the enzyme works well below the maximum reaction rate. They try to compensate for this by accumulating high amounts of the enzyme in their chloroplasts. It has been calculated that at 30 ° C a C 4 leaf needs about 13-20% of the amount of RubisCO on a C 3 leaf to achieve the same rate of photosynthesis (at saturated light intensity). It must be noted, however, that typical C 4 enzymes - such as PPDK and PEPC - result in an increased need for nitrogen. Overall, it is estimated that the so-called nitrogen use efficiency (NUE, nitrogen use efficiency ) in C 4 plants is at least twice as high as in C 3 plants. Increasing attention also gain tropical C 4 -Futtergräser associated with nitrogen-fixing bacteria associated are and therefore hardly an additional fertilization require.
The active increase in carbon dioxide affects the CO 2 saturation curve and the CO 2 compensation point. The latter is far lower in C 4 plants with 0.0005 volume% or less than in C 3 plants with 0.0100 volume%. Falling CO 2 concentrations therefore favor C 4 plants; For example, if you grow a C 3 and a C 4 plant in a closed container under permanent light, both will compete for carbon dioxide. The C 4 plant does this better, so that it actually causes the C 3 plant to die. In contrast, an increase in the CO 2 concentration does not result in an increase in the photosynthetic capacity of C 4 plants. Rather, increasing CO 2 values in the atmosphere favor C 3 plants. This is because, in contrast to the C 4 metabolism, the C 3 metabolism is always dependent on the diffusion rate of the carbon dioxide. Amaranthus palmeri has the highest rate of photosynthesis in the world, this is 80 µmol CO 2 · m −2 · s −1 with a carbon dioxide concentration of 325 µmol · mol −1 CO 2 (in comparison, maize has a rate of 60 µmol · m −2 · s - 1 , for wheat and rice these are 35 µmol · m −2 · s −1 ).
C 4 plants are most C 3 superior plants in that they can be used more economically their Kohlenstoffdioxidanreicherung water (WUE, water use efficiency , dt .: water use efficiency ): The optimum growth temperature is between 30 and 40 ° C, for C 3 -Plants on the other hand at 20 to 30 ° C. The herbaceous C 4 plant Tidestromia oblongifolia found in Death Valley reaches its photosynthesis maximum at temperatures of 45 to 50 ° C. C 4 plants can largely, but not completely, close their stomata over a longer period of time in order to reduce water loss without endangering the carbon balance. While C 4 plants need 230 to 250 ml of water to form 1 g dry matter, the need for C 3 plants is two to three times as high.
Because of the CO 2 pump, the energy requirement of C 4 plants is higher than that of C 3 plants. In the case of the NADP-ME type and NAD-ME type, for example, it is 5 ATP and 2 NADPH per fixed CO 2 molecule. C 3 plants require 3 ATP and 2 NADPH per fixed CO 2 molecule, these values disregarding the photorespiratory losses. C 4 plants of the PEPCK type require 3.6 ATP and 2.3 NADPH per fixed CO 2 molecule. However, through increased cyclic photophosphorylation (photosystem I), ATP can also easily be made available. Due to the increased energy requirement, C 4 plants require more light compared to C 3 plants. This explains why they are preferred in very sunny locations. The light compensation point is also higher compared to C 3 plants, but there is no saturation under natural light conditions.
In cool and cold climates, however, there are very few C 4 plants and are apparently inferior to the C 3 plants. At first it was postulated that the C 4 metabolism is poorly adapted to cold, while it shows advantages at high temperatures. Physiological reasons were first listed for this, for example because of the lability of the enzymes involved in the C 4 metabolism. Another theory assumes that all C 4 plants originated from C 3 plants that have adapted to hot and dry climates. Therefore, the resulting plants could no longer spread in cold regions. Since some C 4 plants have nevertheless developed a certain tolerance to cold, cold in itself does not seem to be an insurmountable barrier. However, those plants still need warm or saline microhabitats , for example southern alpine slopes, which receive sufficient sunlight during the day and are therefore warm enough.
Economic aspects
C 4 plants can be used to produce biomass for energy . Chinese reed achieves yields of 15 to 25 tons of dry matter per hectare and year. In the USA corn is used as the basis for biofuel , in Brazil it is mainly sugar cane . Alternatively, cold-tolerant C 4 grasses such as switchgrass for producing cellulosic ethanol discussed. Under artificially optimized conditions, for example through adequate irrigation, the productivity rates of C 4 plants can generally be increased. Corn or sugar cane plantations, if sufficiently fertilized and irrigated, are among the most productive agricultural ecosystems.
One problem of the growing world population ( overpopulation ) is the shortage of food supplies, especially since less and less land will be available for agricultural use. One way to increase crop yields could be through C 4 photosynthesis. It is particularly advantageous in warmer regions of the world, since there C 3 plants, such as rice , for example , are always inferior to C 4 plants in terms of their photosythesis productivity. One approach for this is already present in nature, but not cultivable C 4 plants, such as barnyardgrass similar rice-grain to grow . Chicken millet uses a C 4 photosynthesis of the PEPCK type.
Alternatively, there are considerations and attempts to increase the photosynthesis rate in C 3 plants by introducing different genes from C 4 plants, for example in rice ( “C 4 rice” ). So far, they have not been very promising. One reason why rice or close relatives could not produce C 4 photosynthesis is that there is only one C 4 -related PEPC enzyme. However, this is specifically involved in the ammonium assimilation in the chloroplast, so that it cannot be used for another task. This also hampers an evolutionary expression in the direction of C 4 photosynthesis.
In principle, there are two ways of transforming a C 3 plant into a C 4 plant and thus increasing productivity:
- Either one starts from a single-cell model in which the C 4 photosynthesis does not take place in two different cells as usual, but within the same cell. In nature, too, there are plants that manage the spatial separation between CO 2 pre-fixation and the Calvin cycle in one and the same cell (see section above ). For the conversion one would have to carry out different steps in order to bring C 4 -specific properties into the cell. So should u. a. the carboxylation enzyme PEPC will be present in the cytosol, the carbonic anhydrase activity of chloroplasts will be transferred to the cytosol, efficient transporters will be introduced into the chloroplast membrane and a spatial separation of PEPC and RuBisCO will be achieved.
- Or one starts from a two-cell model in which an attempt is made to achieve an anatomical specialization with a coronal anatomy, as is the case with most C 4 plants. However, this would require much more genetic interventions than the single-cell model, especially since a new specialized cell type would have to be formed.
Isotope Discrimination
C 4 plants can be recognized by the ratio of the two carbon isotopes 12 C and 13 C. The two isotopes occur in the earth's atmosphere with 98.89% and 1.11% (the radioactive isotope 14 C does not play a role in this context). Although the chemical properties of 12 CO 2 and 13 CO 2 are the same, RuBisCO reacts faster with the lighter carbon isotope. The reason is, on the one hand, that the heavier 13 CO 2 diffuses more slowly. On the other hand, RuBisCO has an intrinsic discrimination value against 13 CO 2 . In C 3 plants, this isotope discrimination means that the 12 C / 13 C ratio is higher than in the atmosphere.
The ratio is expressed by a quotient, the δ- 13 C value:
A certain limestone is defined as the standard ( Pee Dee Belemnite ), the value in the sample is determined by mass spectrometry . Products of the C 3 photosynthesis have δ- 13 C values averaging −28 ‰.
The PEP carboxylase prefers 12 CO 2 less strongly than RubisCO, but in C 4 plants almost all of the CO 2 is pre- fixed by the PEP carboxylase. Due to the high internal CO 2 concentration in the bundle sheath cells, the discrimination against RubisCO does not come into play either. This results in a δ- 13 C value of −14 ‰ on average for C 4 plants .
By determining the δ- 13 C value by means of mass spectrometry, one can therefore distinguish the photosynthetic products from C 3 and C 4 plants, which is widely used. In the food analysis, the origin of the sugar ( sucrose ) can be determined, for example whether sugar comes from sugar beet (C 3 ) or from sugar cane (C 4 ) or whether honey has been "stretched" with cane sugar . By analyzing the (fossil) samples of fat, bones or teeth from animals, conclusions can be drawn as to whether and when they have used pastureland with, for example, C 4 plants. This can also be combined with studies from soil samples, so that historical changes in vegetation, such as a change from C 3 to C 4 grasses, can be documented.
Comparison between C 3 , C 4 and CAM plants
feature | C 3 | C 4 | CAM |
---|---|---|---|
Perspiration quotient [ml (H 2 O) per g (C)] | 450-900 | 250-350 | 18–100 (during the night) or 150–600 (during the day) |
Water use efficiency (dry weight produced in g per g water loss) | 1.05-2.22 | 2.85-4.00 | 8.0-55.0 |
maximum photosynthesis rate [µmol fixed CO 2 / leaf area m 2 · second] | 20-40 | 30-60 | 5–12 (in the light) or 6–10 (in the dark) |
Temperature optimum | 15-25 ° C | 30-47 ° C | 35 ° C |
Gain in dry matter ([tons / hectare · year]) | 10-25 | 40-80 | 6-10 |
δ- 13 C values | −32 to −20 ‰ | −17 to −9 ‰ | −17 to −9 ‰ (drought) or −32 to −20 ‰ (well supplied with H 2 O) |
evolution
Appearance and spread of the first C 4 plants
The first appearance of C 4 plants is still the subject of research. Various techniques are used for dating, such as DNA analyzes ( phylogenetic studies), geochemical signals (e.g. the isotope ratio of 12 C and 13 C), fossils and microfossils ( pollen , phytolites). The C 4 metabolism arose up to 61 times independently of one another in 19 plant families.
There is increasing evidence that the origin of C 4 photosynthesis during the Oligocene is about 30 million years before our time. Falling temperatures and carbon dioxide concentrations (from around 1000 ppm to below 500 ppm) characterize this period. Ten million years ago, carbon dioxide levels continued to drop below 300 ppm. In addition, the atmospheric O 2 concentration rose from 18% to today's 21%. The resulting unfavorable ratio of oxygen to carbon dioxide for C 3 photosynthesis (high photorespiration) is seen as the primary evolutionary pacemaker for the development of CO 2 -concentrating measures in plants, which promoted the development of the C 4 metabolism and CAM. Presumably there was initially a high selection pressure in aquatic plants , which had led to a C 4 metabolism or CAM. In addition, the climatic changes meant that warmer areas in particular became more arid, which must have further favored the selection for C 4 and CAM plants.
Of around 8,100 C 4 plant species from 19 angiosperm families , around 80% are grasses and sour grasses (as of 2017). The approx. 5,000 grasses include the oldest C 4 plants ( Chloridoideae ), but there are also phylogenetically very young species such as Neurachne . Almost 1,300 species belong to the sour grass family that developed from six clades . The remaining C 4 plants belong to the eudicotyledons (around 1,800 species from 16 families). However, C 4 grasses did not necessarily originate before the C 4 eudicotyledons, as both groups contain very old and very new lineages.
Falling CO 2 concentrations are considered to be an evolutionary trigger and general basic requirement for the development of C 4 plants. Since the C 4 photosynthesis also originated during the 30 million years after the first evolutionary spurt, other locally prevailing factors must have played a major role. There are six global centers that are considered to be the nucleus for many C 4 eudicotyledons and some grasses: (Southwestern) North and (Central) South America , South Africa , Northeast Africa and Arabia , Western and Central Asia and Australia . These regions are usually warm and dry to semi-arid but experience regular rainfall during the summer. Also salide , sandy or dry soils favor the emergence and spread of C 4 plants, as they water availability decrease. This would explain why C 4 plants, on the other hand, hardly occur in damp, shaded forests. Another factor are locations with a high incidence of light, as the C 4 metabolism requires more energy. About 23 million years ago, C 4 plants were already widespread in the New World , Africa and South Asia. The spread was gradual, especially in the lower and middle latitudes . A second evolutionary development phase of C 4 plants is located in the early Miocene , during which a further decrease in the CO 2 concentration occurred.
A global, ecological importance resulted from the very strong spread of C 4 grasses, and thus an expansion of the ecosystems dominated by C 4 plants in grasslands and savanna areas . This took place at the end of the Miocene and the beginning of the Pliocene 2 to 8 million years ago. The displacement of C 3 grasses and tree landscapes by C 4 grass landscapes was promoted by increasing aridity and coevolution of large grazing animals (North America) and by regional dry periods and bush fires (Africa, South Asia). This reflects the competition between C 4 grasses and growing (C 3 ) trees, because the saplings of these trees would at some point overgrow and shade the C 4 grass without recurring "disturbances" (for example a bush fire) . After a fire, however, C 4 grasses are able to recover faster than C 3 plants , especially in arid regions . In addition, the rapid growth of many grasses promotes further fires. Therefore, C 4 grasses dominate in areas that have regular dry periods and bush fires, while tree landscapes predominate in humid areas.
Whether or not C 4 photosynthesis took place much earlier cannot yet be conclusively concluded. For example, isotope values from marine sediments in Africa today can be interpreted to mean that the first C 4 plants already existed 90 million years ago. A hypothetically even earlier possible point in time would be the transition from the late Carboniferous to the early Permian about 300 million years ago, since photorespiration had become noticeable due to the prevailing ratio of oxygen to carbon dioxide.
Through phylogenetic studies of C 3 and C 4 plants and C 3 –C 4 intermediate stages, a model has been developed that describes the transition from C 3 to C 4 photosynthesis. This includes several biochemical and anatomical changes and can be summarized in the following overarching development steps:
- Genetic requirements
- Formation of a (proto) crown anatomy
- Establishment of a C 2 photosynthesis
- Development of the complete C 4 dicarboxylic acid metabolism
- further optimizations
Each of the steps involved offers an evolutionary advantage over the last stage of development. This would also explain why the development could take place from different and independent ways. The C 4 metabolism arose neither from a specific biochemical path nor from a certain anatomical structure (only the CA and PEPC reactions have all C 4 plants in common). It is the result of a combination of features, the combined occurrence of which indicates a certain condition - C 4 photosynthesis. Therefore, some authors use in the literature, the term "C 4 - syndrome ".
Basic genetic requirements
The evolution from C 3 to C 4 metabolism is facilitated because many biochemical reactions already occur in a similar form in C 3 plants. However, the enzymes involved take part in reactions that do not necessarily take place in photosynthetically active tissue.
In fact, all of the enzymes involved in C 4 photosynthesis are originally derived from non-photosynthetic isoforms and are involved in other tasks. The regulation of the enzyme required for the new function also changes. This is best documented with PEPC. In contrast to non-photosynthetic PEPC, the “C 4 ” PEPC shows a reduced affinity for PEP, but an increased affinity for bicarbonate. The allosteric inhibition by aspartate and malate is lower, that compared to glucose-6-phosphate and glycine is higher. All changes are based only on minor genetic differences.
A basic requirement for the conversion of enzymes involved in the C 4 metabolism is the presence of redundant genes in the genome, for example as a result of gene duplications. As a result, gene copies of those enzymes can be exposed to various mutations without this being immediately fatal for the plant. These mutations include a cell-specific switching off or an adaptation to the catalytic properties and kinetics of the corresponding enzymes or the development of a coronary anatomy, which is necessary for a C 4 metabolism. A new type of enzyme regulation associated with this is also required. In the case of grasses, the genome duplication that occurred around 70 million years ago could have favored the multiple occurrence of C 4 photosynthesis.
Proto-wreath anatomy
The first step is considered to be the development of the crucial anatomical basis of the C 4 metabolism: the coronary anatomy. This ensures that the transport of pre-fixed carbon dioxide from the mesophyll cells (M) into the bundle sheath cells (BS) can take place as quickly as possible. Ideally, both cell types are in direct contact with one another. One step in this direction is to increase the number of vascular bundles in a (planar) sheet, which also increases the structural integrity of the sheet. This is followed by an “activation” of the BS cells in the second step. Normally, the BS cells of a typical C 3 plant are not photosynthetically active. In addition, the number of its cell organelles is low, since photosynthesis takes place in mesophyll. As a result of the anatomical changes in the direction of C 4 photosynthesis, the BS cells are enlarged and have a larger number of cell organelles, which are associated with increased photosynthetic activity (more chloroplasts) and, with the increasing photorespiratory effects, also form more mitochondria and peroxisomes.
The C 2 metabolism (photorespiratory CO 2 pump)

A third step in the direction of C 4 is the formation of a C 2 photosynthesis (see also section above). For the formation of C 2 photosynthesis it is necessary to reduce the activity of the GDC in the M cells or to switch it off completely. This is usually done by inactivating one of its four subunits. It has been shown in the field morikandia ( Moricandia arvensis ) that the P subunit of the GDC complex is missing in the M cells. As a result, the GDC complex is inactive there. In the C 3 predecessor Moricandia moricandioides, on the other hand, functional GDC complexes are found in both the M and BS cells. In general, the genes for all GDC subunits in C 2 plants are read specifically or at least preferably in BS cells. With the formation of C 2 photosynthesis, the differentiation of BS cells probably continued to advance (further increase in the number of organelles). The shift of GDC activity to the BS is seen as a key step in the C 4 evolution. Furthermore, in this developmental state, photorespiration would no longer be a general disadvantage, since the glycine transported into the BS cells is now an important source of CO 2 there . Therefore, further adjustments (more organelles in the BS cells, optimization of the leaf anatomy) would increase the use of this internal CO 2 source even further.
In addition to the photorespiratory CO 2 pump, the next step in the direction of C 4 photosynthesis could be the increased occurrence of carbonic anhydrase (CA) and PEPC in the cytosol of M cells. In Flaveria bidentis , the original isoform was transported into the chloroplasts, but due to a mutation in a transit protein it remains in the cytosol. CA and PEPC play a key role in pre-fixing CO 2 . Another beneficial side effect is that it is easier to recapture released CO 2 from the neighboring BS cells. For a C 4 photosynthesis, the activity of other enzymes involved in the metabolic pathway, such as. B. the decarboxylating malate enzyme NAD (P) -ME, are increasingly shifted into the BS cells. In return, the PPDK, which regenerates PEP from pyruvate, has to occur more in the chloroplasts of the M cells. Some C 2 plants actually show an increased activity of these enzymes compared to other C 2 and C 3 plants (factor 2-5) - with pure C 4 plants the factor is around 10-50.
Establishment of a C 4 dicarboxylic acid route and optimization
In the last phase of development into C 4 plants, the RuBisCO activity of the M cells is reduced, as otherwise PEPC and RuBisCO compete for the substrate CO 2 . In addition, further optimizations take place in the final phase. Enzymes are further compartmentalized, the light reaction of photosynthesis is adapted, the carbonic anhydrase activity in the M cells is greatly increased and the cell-specific gene expression in the BS and M is promoted. For the PPDK enzyme, for example, a modification of the gene promoter is seen as an important step for a C 4 evolution, as this means that the gene for PPDK is read specifically in the M cells.
The regulation and kinetics of some enzymes, such as PEPC, must be optimized. A high concentration in the mesophyll cell is necessary for the diffusion of malate into the BS cells. However, since malate also inhibits the “original” PEPC, the sensitivity of the C 4 -PEPC to malate must be reduced.
Since many metabolites are transported between two neighboring cells, the formation of a C 4 photosynthesis also requires the necessary transport capacity. All of this enables the efficient transport of CO 2 from the mesophyll cell to the bundle sheath cell.
Reconversion of C 4 to C 3 photosynthesis
An evolutionary reconversion of C 4 to the original C 3 photosynthesis has not yet been proven. One possible reason would be that this requires at least as many evolutionary steps as the reverse route, but no longer promises a selection advantage. A C 3 plant that has been converted back in this way would only have an advantage over C 4 plants in those habitats in which other C 3 plants already dominate. Compared to present C 3 then -Mitbewerbern it could not establish itself.
literature
Review articles and general information
- Agepati S. Raghavendra, Rowan F. Sage (Eds.): C 4 photosynthesis and related CO 2 concentrating mechanisms . Springer, Dordrecht 2011, ISBN 978-90-481-9406-3 (series Advances in photosynthesis and respiration Volume 32).
- Oula Ghannoum: C 4 photosynthesis and water stress . In: Annals of Botany 103 (4), 2009, pp. 635-644, PMID 18552367 , PMC 2707343 (free full text, PDF).
- Donat-Peter Häder : Photosynthesis . 1st edition, Thieme Verlag, Stuttgart 1999. ISBN 978-3-13-115021-9 .
- Hans W. Heldt and Birgit Piechulla: Plant biochemistry . 4th edition. Spektrum Akademischer Verlag, 2008, ISBN 978-3-8274-1961-3 , p. 211 ff .
- Ulrich Lüttge , Manfred Kluge and Gerhard Thiel: Botany - The comprehensive biology of plants . 1st edition, Wiley-VCH Verlag GmbH & Co. KGaA; Weinheim 2010; ISBN 978-3-527-32030-1 .
- Lincoln Taiz (author), Eduardo Zeiger (author) and B. Jarosch (translator): Plant Physiology: The original with translation aids. 4th edition, Spektrum Akademischer Verlag; Heidelberg 2007; ISBN 978-3-8274-1865-4 .
evolution
- Rowan F. Sage: A portrait of the C4 photosynthetic family on the 50th anniversary of its discovery: species number, evolutionary lineages, and Hall of Fame . In: Journal of Experimental Botany . tape 68 , no. 2 , 2017, p. e11 – e28 , doi : 10.1093 / jxb / erx005 , PMID 28110278 .
- RF Sage and Stata, M. (2014): Photosynthetic diversity meets biodiversity: The C 4 plant example . In: J Plant Physiol . pii: S0176-1617 (14) 00236-3; PMID 25264020 ; doi: 10.1016 / j.jplph.2014.07.024 .
- PA Christin and Osborne, CP. (2013): The recurrent assembly of C 4 photosynthesis, an evolutionary tale. In: Photosynth Res . 117 (1-3); Pp. 163-175; PMID 23703454 ; doi: 10.1007 / s11120-013-9852-z .
- RF Sage, TL Sage and Kocacinar, F. (2012): Photorespiration and the evolution of C 4 photosynthesis . In: Annu Rev Plant Biol . 63; Pp. 19-47; PMID 22404472 ; doi: 10.1146 / annurev-arplant-042811-105511 .
- U. Gowik and Westhoff, P. (2011): The path from C 3 to C 4 photosynthesis. In: Plant Physiol. 155 (1); Pp. 56-63; PMID 20940348 ; doi: 10.1104 / pp.110.165308 .
Individual evidence
- ↑ a b c d e f g h i j k l Rowan F. Sage: A portrait of the C4 photosynthetic family on the 50th anniversary of its discovery: species number, evolutionary lineages, and Hall of Fame . In: Journal of Experimental Botany . tape 68 , no. 2 , 2017, p. e11 – e28 , doi : 10.1093 / jxb / erx005 , PMID 28110278 .
- ↑ a b c Andreas Bresinsky and others: Strasburger - Textbook of Botany. 36th edition. Spectrum Academic Publishing House; Heidelberg 2008; ISBN 978-3-8274-1455-7 , p. 312.
- ↑ Hans W. Heldt, Birgit Piechulla: Plant biochemistry . 4th edition. Spectrum Academic Publishing House, Heidelberg 2008; ISBN 978-3-8274-1961-3 , p. 226.
- ↑ a b c d Peter Raven, Ray F. Evert, Susan Eichhorn: Biology of plants . 4th edition. de Gruyter, Berlin / New York 2006, ISBN 3-11-018531-8 , pp. 154 .
- ^ Andreas Bresinsky and others: Strasburger - Textbook of Botany. 36th edition. Spectrum Academic Publishing House; Heidelberg 2008; ISBN 978-3-8274-1455-7 , p. 311.
- ^ A b c Rowan F. Sage, Ferit Kocacinar, David S. Kubien: C 4 photosynthesis and temperature . In: Raghavendra, Sage (Ed.): C 4 photosynthesis and related CO 2 concentrating mechanisms. 2011, pp. 161-195.
- ^ WJ Bond, FI Woodward, GF Midgley: The global distribution of ecosystems in a world without fire . In: New Phytologist . 165, No. 2, 2005, pp. 525-538. doi : 10.1111 / j.1469-8137.2004.01252.x . PMID 15720663 .
- ↑ Elizabeth A. Kellogg: C4 photosynthesis . In: Current Biology . 23, No. 14, August, pp. R594-R599. doi : 10.1016 / j.cub.2013.04.066 .
- ↑ Dietmar Brandes: Are the C 4 plants spreading in Central Europe? , Series for Vegetation Studies 27 - Sukopp-Festschrift, 1995, pp. 365–372.
- ↑ a b c d Robert T. Furbank: Walking the C 4 pathway: past, present, and future . In: J Exp Bot . 67, No. 14, July 2016, pp. 4057-4066. doi : 10.1093 / jxb / erw161 . PMID 27059273 .
- ↑ Hodge, AJ, McLean, JD. and Mercer, FV .: Ultrastructure of the lamellae and grana in the chloroplasts of Zea mays . In: J Biophys Biochem Cytol. . 1, No. 6, November 1955, pp. 605-614. PMID 19866553 . PMC 2223833 (free full text).
- ↑ a b Hatch, MD. (2002): C 4 photosynthesis: discovery and resolution. In: Photosynth Res. 73 (1-3); Pp. 251-256; PMID 16245128 ; doi: 10.1023 / A: 1020471718805 .
- ↑ Kortschak, HP., Hartt, CE. and Burr, GO .: Carbon Dioxide Fixation in Sugarcane Leaves . In: Plant Physiol. . 40, No. 2, March 1965, pp. 209-213. PMID 16656075 .
- ↑ Osmond, CB .: Beta-carboxylation during photosynthesis in Atriplex . In: Biochim Biophys Acta. . 141, No. 1, June 1967, pp. 197-199. doi : 10.1016 / 0304-4165 (67) 90264-4 . PMID 6069308 .
- ^ Hatch, MD. (1971): Mechanism and function of C4 photosynthesis . In: MD Hatch, CB Osmond and Slatyer RO (eds): Photosynthesis and Photorespiration . Wiley-Interscience, New York, pp. 139-152.
- ↑ a b c d e f g h i j k l m n U. Gowik and Westhoff, P. (2011): The path from C 3 to C 4 photosynthesis. In: Plant Physiol. 155 (1); Pp. 56-63; PMID 20940348 ; doi: 10.1104 / pp.110.165308 .
- ↑ Peter Schopfer and Axel Brennicke: Plant Physiology . 7th edition. Spectrum Academic Publishing House; Heidelberg 2010; ISBN 978-3-8274-2351-1 ; P. 281.
- ↑ Root gene boosts photosynthesis , flora research.de , accessed on October 28, 2014.
- ↑ a b Andreas Bresinsky and others: Strasburger - Textbook of Botany. 36th edition. Spectrum Academic Publishing House; Heidelberg 2008; ISBN 978-3-8274-1455-7 , p. 308.
- ↑ a b Ulrich Lüttge, Manfred Kluge and Gerhard Thiel: Botany - A comprehensive biology of plants . 1st edition, Wiley-VCH Verlag GmbH & Co. KGaA; Weinheim 2010; ISBN 978-3-527-32030-1 ; Pp. 776-777.
- ↑ a b Donat-Peter Häder: Photosynthesis. 1st edition, Thieme Verlag, Stuttgart 1999, ISBN 978-3-13-115021-9 , p. 205.
- ↑ a b c Andrea Bräutigam and Andreas PM Weber: Transport Processes: Connecting the Reactions of C 4 Photosynthesis: In: Agepati S. Raghavendra, Rowan F. Sage (Ed.): C 4 photosynthesis and related CO 2 concentrating mechanisms . Springer, Dordrecht 2011, ISBN 978-90-481-9406-3 (series Advances in photosynthesis and respiration Volume 32).
- ↑ a b c Hans W. Heldt, Birgit Piechulla: Plant biochemistry . 4th edition. Spectrum Academic Publishing House, Heidelberg 2008; ISBN 978-3-8274-1961-3 , p. 217 ff.
- ↑ Donat-Peter Häder: Photosynthesis. 1st edition, Thieme Verlag, Stuttgart 1999, ISBN 978-3-13-115021-9 , p. 210.
- ↑ a b c d e Gerald E. Edwards, Elena V. Voznesenskaya: C 4 Photosynthesis: Kranz forms and single-cell C 4 in terrestrial plants. In: Agepati S. Raghavendra, Rowan F. Sage (Ed.): C 4 photosynthesis and related CO 2 concentrating mechanisms . Springer, Dordrecht 2011, ISBN 978-90-481-9406-3 ( Advances in photosynthesis and respiration series, Volume 32), pp. 29-61.
- ↑ Hans W. Heldt, Birgit Piechulla: Plant biochemistry . 4th edition. Spectrum Academic Publishing House, Heidelberg 2008; ISBN 978-3-8274-1961-3 , p. 220.
- ↑ Donat-Peter Häder: Photosynthesis. 1st edition, Thieme Verlag, Stuttgart 1999, ISBN 978-3-13-115021-9 , p. 207.
- ↑ a b c d e f g h i j k l R. F. Sage, TL Sage and Kocacinar, F. (2012): Photorespiration and the evolution of C 4 photosynthesis . In: Annu Rev Plant Biol . 63; Pp. 19-47; PMID 22404472 ; doi: 10.1146 / annurev-arplant-042811-105511 .
- ↑ Hans W. Heldt, Birgit Piechulla: Plant biochemistry . 4th edition. Spectrum Academic Publishing House, Heidelberg 2008; ISBN 978-3-8274-1961-3 , pp. 224-225.
- ↑ a b Chris J. Chastain: Structure, Function, and Post-translational Regulation of C 4 Pyruvate Orthophosphate Dikinase . In: Agepati S. Raghavendra (Eds.) And Rowan F. Sage (Eds.): C 4 Photosynthesis and Related CO 2 Concentrated Mechanisms (Advances in Photosynthesis and Respiration). Springer Netherlands 2011; ISBN 978-90-481-9406-3 ; Pp. 301-315.
- ↑ a b Chris J. Chastain et al. (2011): Functional evolution of C 4 pyruvate, orthophosphate dikinase . In: J Exp Bot 62; Pp. 3083-3091; doi: 10.1093 / jxb / err058 .
- ↑ H. Akhani. et al. (2012): A new species of Bienertia (Chenopodiaceae) from Iranian salt deserts: a third species of the genus and discovery of a fourth terrestrial C4 plant without Kranz anatomy . In: Plant Biosystems 146: 550-559; doi: 10.1080 / 11263504.2012.662921 .
- ↑ George Bowes: Single-cell C 4 photosynthesis in aquatic plants. In: Raghavendra, Sage (Ed.): C 4 photosynthesis and related CO 2 concentrating mechanisms. 2011, pp. 63-80.
- ↑ P. Wang, D. Vlad and Langdale JA (2016): Finding the genes to build C4 rice. Curr Opin Plant Biol. 31; 44-50. doi: 10.1016 / j.pbi.2016.03.012 ; PMID 27055266
- ↑ Hans Lambers, F. Stuart Chapin III. and Thijs L. Pons: Plant Physiological Ecology . 2nd edition, Springer, Berlin 2008; ISBN 978-0-387-78340-6 ; P. 80.
- ↑ Sage, RF. (2002): Are crassulacean acid metabolism and C4 photosynthesis incompatible? In: Functional Plant Biology 29 (6); Pp. 775-785; doi: 10.1071 / PP01217 .
- ↑ Stanislav Kopriva: Nitrogen and Sulfur Metabolism in C 4 Plants In: Agepati S. Raghavendra, Rowan F. Sage (eds.): C 4 photosynthesis and related CO 2 concentrating mechanisms . Springer, Dordrecht 2011, ISBN 978-90-481-9406-3 (series Advances in photosynthesis and respiration Volume 32).
- ↑ Ulrich Lüttge, Manfred Kluge and Gerhard Thiel: Botany - The comprehensive biology of plants . 1st edition, Wiley-VCH Verlag GmbH & Co. KGaA; Weinheim 2010; ISBN 978-3-527-32030-1 ; Pp. 781-782.
- ↑ a b c Rowan F. Sage et al .: Some like it hot: the physiological ecology of C4 plant evolution . In: Oecologia . tape 187 , no. 4 , 2018, p. 941-966 , doi : 10.1007 / s00442-018-4191-6 , PMID 29955992 .
- ↑ a b Ulrich Lüttge, Manfred Kluge and Gerhard Thiel: Botany - A comprehensive biology of plants . 1st edition, Wiley-VCH Verlag GmbH & Co. KGaA; Weinheim 2010; ISBN 978-3-527-32030-1 ; Pp. 785-786.
- ↑ a b Michael B. Jones: C 4 species as energy crops . In: Raghavendra, Sage (Ed.): C 4 photosynthesis and related CO 2 concentrating mechanisms . 2011, pp. 379-397.
- ↑ Lincoln Taiz (author), Eduardo Zeiger (author) and B. Jarosch (translator): Plant Physiology: The original with translation aids. 4th edition, Spektrum Akademischer Verlag; Heidelberg 2007; ISBN 978-3-8274-1865-4 , p. 215.
- ↑ Peter Raven, Ray F. Evert, Susan Eichhorn: Biology of plants . 4th edition. de Gruyter, Berlin / New York 2006, ISBN 3-11-018531-8 , pp. 823 .
- ↑ Kerstin Stolzenburg: Test results willows, poplars and miscanthus from LAP Forchheim. (PDF; 348 kB) December 14, 2006, archived from the original on December 11, 2014 ; accessed on August 8, 2016 .
- ↑ Donat-Peter Häder: Photosynthesis. 1st edition, Thieme Verlag, Stuttgart 1999, ISBN 978-3-13-115021-9 , p. 214.
- ^ A b James N. Burnell: Hurdles to engineering greater photosynthetic rates in crop plants: C 4 rice . In: Raghavendra, Sage (Ed.): C 4 photosynthesis and related CO 2 concentrating mechanisms . 2011, pp. 361-378.
- ↑ Website of the C4 rice project ( Memento of the original from February 3, 2017 in the Internet Archive ) Info: The archive link was inserted automatically and not yet checked. Please check the original and archive link according to the instructions and then remove this notice. , accessed February 3, 2017
- ↑ a b c P. A. Christin and Osborne, CP. (2013): The recurrent assembly of C 4 photosynthesis, an evolutionary tale. In: Photosynth Res . 117 (1-3); Pp. 163-175; PMID 23703454 ; doi: 10.1007 / s11120-013-9852-z .
- ↑ Hans W. Heldt, Birgit Piechulla: Plant biochemistry . 4th edition. Spectrum Academic Publishing House, Heidelberg 2008; ISBN 978-3-8274-1961-3 , p. 225.
- ↑ Lincoln Taiz (author), Eduardo Zeiger (author) and B. Jarosch (translator): Plant Physiology: The original with translation aids. 4th edition, Spektrum Akademischer Verlag; Heidelberg 2007; ISBN 978-3-8274-1865-4 , pp. 216-217.
- ↑ Lincoln Taiz (author), Eduardo Zeiger (author) and B. Jarosch (translator): Plant Physiology: The original with translation aids. 4th edition, Spektrum Akademischer Verlag; Heidelberg 2007; ISBN 978-3-8274-1865-4 , p. 217.
- ↑ Peter Schopfer and Axel Brennicke : Plant Physiology . 7th edition, Spektrum Akademischer Verlag; Heidelberg 2010; ISBN 978-3-8274-2351-1 , p. 295.
- ^ Andreas Bresinsky and others: Strasburger - Textbook of Botany. 36th edition. Spectrum Academic Publishing House; Heidelberg 2008; ISBN 978-3-8274-1455-7 , p. 1012.
- ^ Ulrich Lüttge, Manfred Kluge, Gabriela Bauer: Botany . 5. completely revised Edition. Wiley-VCH, Weinheim 2005; ISBN 978-3-527-31179-8 ; P. 485.
- ↑ Caroline Bowsher, Martin W. Steer, Alyson K. Tobin: Plant Biochemistry . Garland Pub, New York, NY 2008, ISBN 978-0-8153-4121-5 ; P. 136.
- ↑ Rowan F. Sage: The evolution of C4 photosynthesis . In: New Phytologist . 161, No. 2, February 1, 2004, ISSN 1469-8137 , pp. 341-370. doi : 10.1111 / j.1469-8137.2004.00974.x .
- ↑ RF Sage and Stata, M. (2014): Photosynthetic diversity meets biodiversity: The C4 plant example . In: J Plant Physiol . pii: S0176-1617 (14) 00236-3; PMID 25264020 ; doi: 10.1016 / j.jplph.2014.07.024 .
- ↑ a b c Jens Boenigk: Biodiversity and Earth History. Springer-Verlag, 2015, ISBN 978-3-642-55389-9 , p. 144.
- ↑ a b Ulrich Lüttge, Manfred Kluge and Gerhard Thiel: Botany - A comprehensive biology of plants . 1st edition, Wiley-VCH Verlag GmbH & Co. KGaA; Weinheim 2010; ISBN 978-3-527-32030-1 ; P. 797.
- ^ PA Christin. et al. (2011): C 4 eudicots are not younger than C 4 monocots In: J. Exp. Bot. 62 (9): pp. 3171-3181; doi: 10.1093 / jxb / err041 .
- ↑ a b c Hans Konrad Biesalski: Micronutrients as the engine of evolution. Springer-Verlag, 2015, ISBN 978-3-642-55397-4 , p. 63.
- ^ EJ Edwards, SA Smith: Phylogenetic analyzes reveal the shady history of C4 grasses. In: Proceedings of the National Academy of Sciences . Volume 107, number 6, February 2010, pp. 2532-2537, doi: 10.1073 / pnas.0909672107 , PMID 20142480 , PMC 2823882 (free full text).
- ^ Colin P. Osborne: The geologic history of C 4 plants. In: Raghavendra, Sage (Ed.): C 4 photosynthesis and related CO 2 concentrating mechanisms . 2011, pp. 339-357.
Web links
- Axel Brennicke: The evolution of C 4 photosynthesis: How slightly modified C 4 pathways could arise almost 50 times in parallel. In: BiuZ. 6/2011, pp. 362-363.
- Herfried Kutzelnigg: The evolution of the C 4 plants. In: Studium Integrale Journal. Volume 15 / Issue 1 - April 2008, accessed on October 28, 2014.