Virus envelope
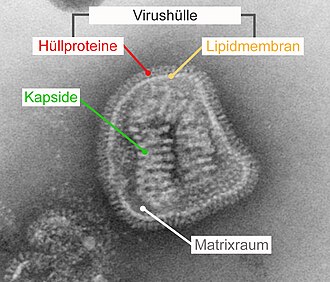
The viral envelope ( English viral envelope ) is in certain viruses existing external structure of lipids of a lipid double membrane of the original host cell and incorporated therein viral proteins exist. The virus envelope usually encloses a capsid in which the viral nucleic acid is packaged. Depending on the type of virus, the envelope arises from the cell membrane on the cell surface or from membranes of the endoplasmic reticulum (ER) or Golgi apparatus inside the cell.
The presence of a virus envelope is an important criterion in the classification of viruses, the so-called virus taxonomy . The enveloped viruses are distinguished from the non-enveloped or "naked" viruses. While non-enveloped viruses always have to leave the infected cell by destroying the host cell, enveloped viruses can be released without such a lysis . The virus envelope is of great importance for the uptake of viruses into the cell, the stability against environmental influences and disinfectants as well as the facilitated ability to change the virus surface. This variability through a virus envelope is an evolutionary advantage over non-enveloped viruses. It enables enveloped viruses to subvert a host's immune defenses more easily or to adapt better to a new host. These properties of the virus envelope become clear, for example, from the fact that all viruses that appear in humans ( emerging viruses ) that represent a real or potential threat from a pandemic are enveloped viruses. B. the HI virus , SARS coronavirus 1 and 2 , influenza virus , Ebola virus and West Nile virus .
discovery
The beginnings of virology and the definition of viruses as a new type of infectious pathogen are linked to two non-enveloped viruses: the tobacco mosaic virus ( Dmitri Iwanowski 1892 and Martinus Beijerinck 1898) and the foot-and-mouth disease virus ( Friedrich Loeffler and Paul Frosch 1897). The yellow fever virus discovered by Walter Reed in 1901 was the first virus identified in humans and at the same time the first enveloped virus described. However, these investigations were limited to transmission routes, the morphology of the viruses remained initially unknown apart from the property of being particularly small (invisibility in the light microscope ).
This barrier of insufficient microscopic resolution could only be overcome in the 1930s with the development of the electron microscope by Helmut and Ernst Ruska . Even the first recordings with this new technology showed the outlines of viruses with an elongated or round shape. A differentiation of the fine structure of the viruses and representation of the virus envelope was not yet possible with the early contrast staining. After all, in 1943, after examining the virus isolates available at the time, Helmut Ruska proposed an initial classification of viruses according to size and shape. Until then, the viruses were classified according to the infected host and the respective disease.
In the 1950s, viruses could also be specifically grown in the cell cultures developed by Renato Dulbecco and Harry Eagle and propagated in large quantities. The purity and concentration of this virus preparation made it possible to more precisely determine the chemical composition and thus the lipid content of viruses. Until this technique was established, one had to limit oneself to virus isolation from infected hosts or to the culture in incubated chicken eggs developed in 1932 and improved in 1946 for virus reproduction . Some viruses lost their ability to infect the chicken embryos if the virus solution was treated with various substances, including fat-dissolving compounds such as ethers ( diethyl ether ) or detergents such as sodium deoxycholate . This so-called "ether sensitivity" of viruses was only observed with some viruses such as the influenza viruses or the herpes viruses , others such as the poliovirus or the foot-and-mouth disease virus were still infectious even after treatment with ether. The sensitivity to ether thus became a further important criterion in the classification of viruses and in the 1950s could already be associated with the detection of lipids in purified viruses. Ether-sensitive viruses had a lipid content of 20-30%.

It was already suspected at the time that the lipid portion of the virus could be related to a membrane structure. The existence of lipid-containing double membranes in cells could already be proven by the studies of Gorter and Grendel in 1925, and it was obvious to assume a similar structure in lipid-containing viruses. The decisive factor was the evidence that the composition of the lipid components of the viruses was similar to that of the host cells in which the viruses were grown. The first evidence of a virus envelope in electron microscopic images can be traced in retrospect in a study by Coriell in 1950. He isolated herpes simplex viruses from cold sores. He observed a peculiar, round shape of the virus with a central recess, which he described as " donut- like". Today this typical appearance of the herpes viruses is called "fried egg shape", this means an icosahedral capsid inside surrounded by a very thick virus envelope. It was not until 1959, when a special contrasting process with uranium salts was developed for electron microscopy, that the structure of the viruses became much more differentiated, so that the virus envelope could also be made visible. This so-called negative contrast staining is still the most important method for the electron microscopic visualization of viruses.
The exploration of cellular membranes in the 1960s and 1970s was accompanied by an expansion of our understanding of the virus envelope. This was made possible by refined techniques for structure elucidation of the envelope proteins such as X-ray diffraction , freeze fracture SEM and NMR spectroscopy , but also thanks to new considerations about the properties of biomembranes such as the liquid mosaic model by Singer and Nicholson. In the last twenty years, cryo-electron microscopy in particular has provided crucial insights into the fine structure of the virus envelope. With this technique it is possible to determine the shape and arrangement of individual envelope proteins and to display the virus envelope with a resolution of 0.6–1 nm using Fourier-based image processing.
Structure of the virus envelope
A virus envelope always consists of viral envelope proteins that are embedded in a lipid double membrane. The envelope proteins are deposited in the membrane during their synthesis on the ribosomes of the rough endoplasmic reticulum (rER). Either the virus envelope can already form here from the membrane of the rER or the membrane areas occupied by envelope proteins are transported to the cell membrane, nuclear membrane or the Golgi apparatus by the normal cellular membrane flow. Because the envelope proteins concentrate and accumulate in smaller membrane areas during the coating process, cellular membrane proteins are displaced, which are then not incorporated into the virus envelope. Because of this displacement of cellular membrane proteins, the double membrane of the virus envelope does not consist of unchanged cellular membranes, but only of their lipid content.
The proportion of stored coat proteins is usually so high that the lipid proportion is not uncovered at any point on the surface. The lipid membrane of the virus envelope is therefore no longer directly accessible to antibodies . In some viruses, such as the Hepadnaviridae , the protein content of the virus envelope is so high that the virus envelope consists almost exclusively of tightly packed envelope proteins. These are arranged very regularly and are more resistant to environmental influences and detergents than other enveloped viruses.
Lipid content

The lipid membrane of the virus envelope, like all cellular membranes, consists of a double layer of phospholipids . These have a hydrophilic head, which forms the surface of the membrane, and two inwardly directed, lipophilic hydrocarbon chains . The phospholipids involved in the structure of the virus envelope are phosphatidylcholines (also called lecithins ), phosphatidylethanolamines , phosphatidylserines , phosphatidylinositol and sphingomyelins . The latter are only present in the outer layer of the double membrane. In addition to the phospholipids, there is also a variable proportion of cholesterol . The cellular membranes, and thus also the virus envelopes, vary in the composition of the various phospholipids and the cholesterol content. A high cholesterol content is typical for the cell membrane, while the membranes of the endoplasmic reticulum and the Golgi apparatus contain only a small amount of cholesterol. The cholesterol content of a membrane, expressed as the C / P quotient ( molar cholesterol / phospholipid quotient ), has a decisive influence on the morphology of a membrane, so are cholesterol-rich membranes (i.e. with a typical C / P quotient of 0.4 to 0.8 ) more stable, less flexible and, at 5–6 nm, around a third thicker than low-cholesterol. Since the lipid composition of a virus envelope corresponds in a first approximation to that of the original cellular membrane, these differences can also be found between virus envelopes which are derived from the cell membrane or from intracellular membrane systems.
On closer inspection, the lipid composition of most virus envelopes deviates to a small extent from their original membrane. How this selective absorption of lipid components into the virus envelope takes place is still unclear. A preferred incorporation of various phospholipids during the aggregation of coat proteins in the membrane is assumed, the coat proteins interacting with the lipids to different degrees and the binding of the coat proteins to one another preferring certain phospholipids. The discovery of so-called lipid rafts , i.e. micro-areas with a high cholesterol content floating in a cellular membrane, has shown an inhomogeneous structure of these membranes. These lipid rafts also appear to be important for selective incorporation into the virus envelope. In the case of some viruses, the presence of these micro-areas is even a necessary prerequisite for the storage of the envelope proteins and the formation of the virus envelope, since they increase the density of viral envelope proteins regionally and thus enable aggregation. Conversely, the presence of cholesterol in the viral envelope of some viruses is necessary for penetration into the cell. Growing the canine distemper virus in cell cultures to which an inhibitor for cholesterol synthesis was added reduced its ability to infect other cells by 80%. The same was also observed in the case of the varicella-zoster virus envelopes that do not arise on the cholesterol-rich cell membrane.
Table: Comparison of the lipid components of typical cellular membranes (rER: rough ER, sER: smooth ER) and virus envelopes ( human immunodeficiency virus HIV-1, spherical antigen particles of the hepatitis B virus sHBV). For better comparability, the lipid components are each converted into molar percent of the lipid fraction for the rat liver cell from the data of MK Jain (1980). The sER membrane also contains 2.0% diphosphatidylglycerin ( cardiolipin ).
Lipid component | Cell membrane | rER membrane | sER membrane | Golgi membrane | HIV-1 | sHBV |
---|---|---|---|---|---|---|
cholesterol | 34.5 | 6.6 | 10.4 | 9.1 | 46.8 | 3.1 |
Phosphatidylcholine | 20.7 | 60.4 | 56.9 | 48.8 | 12.7 | 78.9 |
Sphingomyelin | 16.0 | 3.3 | 12.4 | 12.2 | 15.1 | 1.9 |
Phosphatidylethanolamine | 12.6 | 17.6 | 21.7 | 18.3 | 13.1 | 9.2 |
Phosphatidylinositol | 4.6 | 8.8 | 6.9 | 7.3 | 1.1 | 3.6 |
Phosphatidylserine | 10.3 | 3.3 | - | 4.3 | 8.0 | 0.9 |
Lipid content% | 87.0 | 91.0 | 96.6 | 82.0 | 28.0 | 24.0 |
C / P quotient | 0.53 | 0.07 | 0.11 | 0.10 | 0.88 | 0.03 |
As can be seen from a comparison of the lipid components listed in the table above, by determining the lipid composition of a virus envelope, conclusions can be drawn about the cellular membrane of origin. In the example given, HIV-1 has the typical composition of the cell membrane and the spherical, empty HBV particles - whose lipid composition corresponds to the complete virions - the lipid profile of the rough ER. The specific enrichment and depletion of the components compared to the original membrane can also be seen. Since the lipid composition of the membranes of different cell types can vary, corresponding deviations in the virus envelope are also to be expected in a virus when it multiplies in different cell types in the organism or in cell culture . Slightly different lipid proportions can also occur within a single cell if the cell membrane has a directed polarity such as e.g. B. in cells that are arranged on a lumen . Different virus envelopes can form in apical or basal areas of the cell membrane.
The importance of the lipid components for the important functions of the virus envelope such as virus uptake, infectivity, membrane fusion and assembly of the virus particles was not recognized for a long time, since the main focus was on researching the envelope proteins. However, many studies on different viruses have recently shown how much the lipids enable the function of the coat proteins through protein-lipid interactions ; In particular, the accumulation of cholesterol in the lipid rafts seems to have a decisive influence on the function of the virus envelope. The lipid membrane has a considerable influence on the arrangement of the envelope proteins and their correct folding as a tertiary structure .
Viral envelope proteins

The viral envelope proteins are incorporated into the lipid membrane in a similar way to cellular transmembrane proteins. One or more transmembrane, lipophilic protein domains cross the lipid membrane and thus separate a smaller inner domain from a larger outer one. Most of the envelope proteins have the carboxyl terminus on the inside, so that the envelope proteins belong to the class 1 membrane proteins.
The inwardly directed domain (also called “intracellular anchor” or anchor domain) is hydrophilic and can mediate the binding to subsequent internal structures. In the classic case, this is a capsid. In viruses with multiple capsids or complex viruses, the inner domain binds to other proteins that also line the underside of the virus envelope. These lie between the capsid and the shell in the matrix space and are therefore referred to as matrix proteins . In the simplest case, the inner domain of the coat protein consists of a folded end of the protein. If the coat protein crosses the lipid membrane several times ( “multipass” ), the inner domain is a resulting loop. The interaction between the inner domains, either directly without further binding partners or indirectly via matrix proteins or capsid, is the determining force for the curvature of the membrane during the envelope.
The transmembrane domain consists of a lipophilic α-helix , the length of which is adapted to the thickness of the lipid membrane. Those viruses that are enveloped in the thicker, cholesterol-rich cell membrane, need for the helix z. B. 26 amino acids ( influenza virus ). If the viruses are enveloped on the membrane of the rER, 18–20 amino acids ( yellow fever virus ) are sufficient for a transmembrane helix. The structure elucidation of an envelope protein may therefore provide an indication of the membrane on which the virions are formed. The viral coat protein can also have several transmembrane domains, the helices of which form bundles lying close together in the membrane. The coat proteins of the Flaviviridae family have two transmembrane helices, the close bond between which is mediated by a hydrophilic flank; these domains thus have an amphiphilic structure. Since the helices displace the lipids of the membrane, the general rule can be that the more transmembrane domains the envelope proteins have, the lower the lipid content of a virus envelope.

The outer part of a coat protein is usually glycosylated in many places, i.e. covalently linked with short sugar residues ( oligosaccharides ), which is why viral coat proteins are counted among the glycoproteins . This outer part of the coat protein is essential for binding to receptors and for membrane fusion during virus uptake. The outer domains are also recognized by antibodies of the immune defense, so that exposed epitopes often contain very variable sections, which are usually referred to as hypervariable regions (HVR). The HVR of the envelope proteins lead to a high immunological flexibility of the virus, since they limit the binding of antibodies through frequent mutations and can quickly adapt to different cell receptors of new hosts .
The tasks of the outer domain - receptor binding and membrane fusion - can be combined in one coat protein or distributed over several cooperating coat proteins. With only a few exceptions, the envelope proteins assemble into complexes made up of several identical or different envelope proteins. If the size of these oligomers is appropriate, they can be seen as so-called “spikes” or peplomers in the electron microscope display . Very characteristic spikes can be seen, for example, in the virus families Orthomyxoviridae and Coronaviridae ; the latter also got their name from this characteristic of the virus envelope.
The number of different envelope proteins and the composition of the envelope protein oligomers is characteristic of many virus genera. The rhabdoviruses have only one coat protein that forms simple trimers ([G] 3 ). In retroviruses , e.g. B. the Rous sarcoma virus , two glycoproteins (SU and TM) combine to form a heterodimer , which in turn arranges itself with two other heterodimers to form a hexamer ([SU-TM] 3 ). Alphaviruses have two (E1, E2) or three envelope proteins (E1-3) which, when assembled, arrange themselves to form larger three-part complexes ([E1-E2-E3] 3 ).
In the case of viruses that bud on the cell membrane, the envelope proteins are first stored in the cell membrane and this is then enriched with proteins that are capable of membrane fusion. This change in the cell membrane can lead to the fact that cell membranes of neighboring cells can fuse with one another through the viral envelope proteins and thus form giant cells, so-called syncytia . This can be advantageous for the virus, since the infection can spread as the cells fuse and a larger synthesis apparatus is available for the viruses; this is e.g. B. the case with respiratory syncytial virus . The fusion of cells in the skin by envelope proteins of the measles virus causes local inflammation , which then becomes visible as the typical reddish spots of a measles infection. However, many viruses have developed strategies to prevent syncytia from forming, as this can also hinder the budding of new viruses. In this case, the fusion properties of the coat proteins are only activated by an additional maturation step, in which the fusion sequence is either only exposed after digestion by a protease or glycosidase or an acidic environment ( pH <6.0) causes a change in the conformation of the coat protein the fusion sequence is first turned outwards. The best-known example is the envelope protein hemagglutinin of the orthomyxoviruses , which first has to be activated by a neuraminidase and has a pH-dependent fusion activity.
Envelope proteins also occasionally fulfill other functions in the cell membrane during virus replication than just enveloping the virion. For this purpose, they can alternatively arrange themselves to form new structures and, as in the case of the SARS coronavirus, for example, form pores that lead to cell lysis .
Symmetrical virus envelopes

The inner portion of the envelope proteins can interact with an enveloped capsid in such a way that only one envelope protein (or an aggregated dimer or trimer of the envelope proteins) binds to only one capsomer . This fixed arrangement transfers the shape and symmetry of the inner icosahedral capsid to the outer virus envelope and, despite the mobility of the lipid membrane, virus envelopes with a strictly icosahedral structure result. This form of so-called " morphogenesis inside out" is found in the genus Alphavirus of the family Togaviridae (eg. As the Semliki Forest Virus and Sindbis virus ) and the genus Flavivirus of the family Flaviviridae .
In the larger viruses of the Bunyaviridae family (80–120 nm), a regular arrangement of the envelope proteins in the form of an icosahedron is also detectable ( triangulation number T = 12), but no symmetrical, icosahedral capsid that could support this symmetry from the inside. Here, the close interaction of the envelope proteins with one another determines the shape of the virus envelope, which can also be referred to as “morphogenesis from the outside in”. With the bunyaviruses, three helical capsids are packed in the virus envelope, which, similar to the influenza viruses, enables the RNA segments to be exchanged ( reassortment ) and a high level of genetic flexibility. This flexibility of the influenza viruses due to several irregular capsids or RNA strands is bought at the price of a disordered and therefore very unstable virus envelope, which can be inactivated by drying out and using mild detergents . The Bunyaviridae family, on the other hand, gives the symmetrical and thus firmer arrangement of the coat proteins a comparatively high level of stability. B. in the genus Hantavirus - remain infectious for months in a dry state and even survive excretion in the urine, although urea acts as a detergent and inactivates other enveloped viruses.
Special forms
In a few virus families, a lipid double membrane is not present as an outer, enveloping structure, but is located inside the virions. Two families of bacteriophages , the Corticoviridae and Tectiviridae , in which the lipid membrane is located inside an icosahedral capsid, are particularly exceptional . This structure is not referred to as the virus envelope, as it neither lies on the outside nor fulfills typical tasks of a virus envelope such as attachment to the cell surface. The membrane vesicle present in the Tectiviridae is used after the capsid has attached to the bacterial surface for the active penetration of the double-stranded bacteriophage DNA into the host cell.
In members of the Poxviridae family , the virus envelope consists of an outer and an additional inner double membrane. Within the cytosol , the smallpox viruses are present with a simple envelope. This first envelope is not created by budding from a cellular membrane, but by assembling a completely new lipid membrane on the outside of the immature, later double- concave capsid. Degraded membrane components from the transition area between Golgi and ER membrane (intermediate compartment) are used to rebuild the membrane. The simply enveloped virus particle is then given a second, outer virus envelope by budding on the Golgi membrane.
Formation during virus replication
The synthesis of the envelope proteins and the formation of the virus envelope mark the last phase in the replication cycle of an enveloped virus; beforehand, the viral genome must be replicated and possibly packaged in a capsid.
The operation of the envelope of a virus, including budding ( "budding" ) called, corresponds to a specific packaging in a pinch-off membrane vesicles. Within cells, the constant formation and fusion of membrane vesicles is a physiological process for substance transport, the so-called exocytosis or endocytosis . An enveloped virus uses these already existing properties and mechanisms of membrane flow by modifying it and controlling it through the viral structural proteins. The energy that is required for the curvature of the lipid membrane and for the formation of bubbles comes exclusively from the interaction of the coat proteins with one another, the coat proteins with internal structures such as matrix proteins and capsids or the capsids with the lipid membrane; a supply of energy, for example in the form of ATP, is not necessary for this. In the case of togaviruses , for example, the energetically more favorable aggregation of the coat proteins overcomes the energetically less favorable curvature for the lipid membrane by means of hydrogen bonds , ionic bonds and especially through hydrophobic interactions . The formation of the virus envelope - regardless of the membrane system - is therefore only controlled by the translation , the transport and the concentration of the virus proteins on the respective membrane compartment. Budding as the spontaneous aggregation of capsid, lipid membrane and coat proteins is of great interest with regard to its thermodynamic consideration. Models were often used to describe them in order to be able to calculate the interaction of the components involved. In agreement with the time measured in vitro that the budding process takes, 10 to 20 minutes could also be calculated in the model. The diffusion of the envelope proteins along the lipid membrane and the displacement of water molecules between envelope and capsid were derived as limiting processes . The model calculations also suggest a preferred budding of viruses at those points of the cellular membrane where there is already a morphological curvature of the lipid membrane. This agrees with the electron microscopic examinations of infected cells in which budding viruses were found predominantly on the curved sides of the Golgi apparatus or the cell membrane.
The mechanisms of the covering are in principle similar on the respective cellular membrane. The first model for budding was developed using the example of the Semliki Forest Virus (SFV). Here, the binding of the intracellular anchor of the envelope proteins to an already closed capsid leads to the curvature of the lipid membrane. From this the thesis was derived that the presence of envelope proteins and the binding to capsid proteins are absolutely necessary for the development of a virus envelope . This early model was considerably restricted when a coating of capsids was observed in retroviruses even without the presence of the envelope proteins ( Env protein ) when the capsid proteins ( Gag proteins ) are available alone in transfected cell cultures.

In addition to the special case for the Gag protein of the retroviruses, there are three other important variants of budding (see illustration). The easiest way is the slight curvature of the lipid membrane due to the interaction of the envelope proteins via their inner anchor domains. A closed capsid binds to these aggregated envelope proteins and, through interaction with the envelope proteins, drives the budding process (see figure, case A). In the second case (B) the assembly of the capsid takes place only after binding to the envelope proteins. The interaction of capsid proteins and envelope proteins enables the nucleic acid to bind and completes the virus during budding. This variant can also be supplemented by a matrix protein mediating between the shell and the capsid. For viruses that do not have symmetrical capsids (for example the bovine virus diarrhea virus and the related hepatitis C virus ), it is sufficient to bind the nucleic acid to basic proteins ( nucleo or core proteins ), which are similar to the matrix proteins on the inside of the membrane the envelope proteins interact.
In the third variant (C), the interaction of the intracellular anchors of the envelope proteins is only made possible by binding to matrix proteins. After this interaction has led to a first curvature of the lipid membrane, a capsid (as with the herpes viruses) or several helical capsids (as with the Orthomyxoviridae ) can be bound and enveloped.
- Formation of the virus envelope through budding: TEM images of infected cell cultures
Aggregation of envelope proteins and curvature of the cell membrane in HIV- 1
Lassa virus in the late phase of the constriction of the virus envelope
Human herpes virus 6 after release on the cell membrane
Enveloped particles of the Rift Valley Fever virus ( Bunyaviridae ) in the lumen of the ER
Bud on the cell membrane
The formation of the virus envelope on the cell membrane first requires the envelope proteins to be transported to the cell surface. The viral proteins arise on the ribosomes of the rough ER, with the envelope proteins penetrating the membrane of the ER with their transmembrane domains during the synthesis and being incorporated into it. The envelope proteins are glycosylated via the membrane system of the Golgi apparatus . The now modified (mature) envelope proteins are transported to the cell membrane in constricted, exocytotic vesicles and fuse with it. Those domains of the envelope proteins that were previously directed into the lumen of the ER are now arranged extracellularly . The remaining virus components (capsids, nucleic acids and possible matrix proteins) brought to the cell membrane can now be enveloped. The path of origin via the cell membrane requires the storage of viral envelope proteins, which can lead to the aforementioned formation of syncytia (see section envelope proteins ). These virus proteins presented to the outside, however, can also be recognized as foreign by immune cells, so that an early immune response against the envelope proteins can take place. All viruses whose envelopes are derived from the cell membrane are also reabsorbed by the fusion of the envelope with the cell membrane. This type of uptake ( fusion from without ) enables infection without transport in an endosome .
Important virus families that are characterized by budding on the cell membrane are, for example, the Retroviridae , Orthomyxoviridae , Togaviridae and all virus families with a single-stranded RNA of negative polarity (ss (-) RNA) as the genome (order Mononegavirales ), i.e. the Bornaviridae , Rhabdoviridae , Filoviridae and Paramyxoviridae .
Bud on the Golgi and ER membrane
Since the envelope proteins are initially always stored in the membranes of the intracellular membrane systems, budding can also take place here. Either the lipid membrane of the ER or - after vesicle transport - the Golgi apparatus can be selected for this development path. This is mainly determined by the possibly necessary modifications of the envelope proteins, which can almost only be carried out by the enzymes of the Golgi apparatus. Very often the budding takes place in the transition area of the two membrane systems, the so-called intermediate compartment . After budding, the enveloped virus particle is always located in the lumen of the membrane system, from where it is transported to the outside inside a transport vesicle ( exosome ). This intracellular envelopment of the virus can take place without destroying the cell, since no virus proteins change the cell surface and normal exocytosis is used to discharge the virus. When the next host cell is infected, all of these viruses must be taken up by an endosome, with the membrane of which the virus envelope fuses ( fusion from within ). Important virus families with intracellular budding are the Coronaviridae , Hepadnaviridae and Flaviviridae .
Bud on the nuclear membrane
The members of the virus family Herpesviridae are a special case in their structure, their reproduction strategy and also in the formation of the virus envelope, since the very large capsids of the herpes viruses are assembled in the cell nucleus , in which the double-stranded DNA of the viruses is also synthesized. Even very early electron microscopic examinations of cells in which the herpes simplex virus multiplies revealed budding capsids on the inside of the nuclear membrane and enveloped virus particles in the perinuclear cistern surrounding the nucleus . Since the perinuclear cistern is connected to the rough ER via membrane tubes, it was assumed that the enveloped virions are then channeled out of the cell via membrane vesicles of the Golgi apparatus. An examination of the lipid composition of the virus envelope showed, however, that the lipid components do not correspond to those of the nuclear membrane, but rather have the lipid profile of the Golgi membrane. This finding led to the discovery that the herpes viruses first acquire a viral envelope by budding on the nuclear membrane. However, this fuses again with the outer membrane of the perinuclear cistern and releases the bare capsid into the cytosol. Only through a second budding in a constricted membrane vesicle of the Golgi apparatus, which is enriched with viral envelope and matrix proteins, the capsid receives its final envelope. This so-called secondary envelope then only corresponds to the virus envelope of the released viruses.
Empty virus envelopes and "defective viruses"
In some viruses, the envelope proteins are able to cause budding without further binding to an internal structure. This is particularly the case when the interaction between the inner anchor domains of the envelope proteins is particularly high. The result is empty or incompletely filled virus envelopes. The existence of these empty shells was first discovered during studies of the so-called "Australia Antigen", which led to the discovery of the hepatitis B virus (HBV) by B. Blumberg . The discovered antigen consists of the three envelope proteins of HBV (HBs antigen). In the blood of people infected with HBV, the HBs antigen is found predominantly in empty, spherical particles with a diameter of 22–24 nm and empty tubular structures (“tubules”) of variable length. Only one infectious, complete virus (42 nm) can be detected out of around 1,000 to 10,000 HBs antigen-containing particles. This huge excess of empty virus envelopes serves primarily to neutralize antibodies against the envelope protein and thus to prevent their binding to the complete virus.
Empty virus envelopes, which, as in the example of HBV, are often smaller than the complete viruses, are also found with incorrect or incomplete packaging of segmented genomes (e.g. influenza virus) when they are grown in cell cultures. These particles are also known as defective interfering particles (DIP) or virus-like particles (VLP). In the case of the hepatitis C virus , the existence of incomplete particles in the blood serum of patients was suspected, since a changing stoichiometric ratio of core protein to RNA can be detected.
The hepatitis D virus provides a special example of the virus envelope , as it does not itself have any genes for adequate packaging with envelope proteins. It depends on the presence of the HBV in the same cell, since it can only be packaged and released with the envelope proteins of the HBV. It is therefore called a defective or dependent virus ( virusoid ).
The capsids of enveloped and non-enveloped viruses
In virus envelopes with a high lipid content, the envelope proteins are arranged flexibly and can move sideways in the membrane. This liquid property of the virus envelope means that a closed envelope is present even if there is an error in the arrangement of the envelope proteins or a gap in the surface symmetry. In the case of non-enveloped viruses, such a disorder would lead to inadequate protection of the genome or to the disintegration of the capsid. Under the protection of a virus envelope, there is greater freedom for the structure of the capsid compared to non-enveloped viruses, since these no longer serve directly to protect the genome from nucleases or represent a point of attack for the immune system. The capsids of enveloped viruses can therefore also have gaps or just cover the genome like a network. This is of great importance for retroviruses and the closely related hepadnaviruses (e.g. the hepatitis B virus ), since the capsid, which is not yet enveloped but closed, can still absorb ATP and nucleotides during replication in order to complete the already packaged genome . In the capsids of some enveloped viruses, the gaps also allow a release of the genome z. B. to the nuclear pore without the capsid having to disintegrate in the cytosol beforehand.
Biological importance
Virus envelope as a pathogenicity factor
As the outer structure, the virus envelope is responsible for all properties of a virion that affect the path of infection, its uptake into the cell and the defense by the immune system. In this confrontation with the host organism mediated by the virus envelope, mechanisms have emerged in the course of viral evolution that are advantageous for the replication of the virus and are referred to as virulence or pathogenicity factors . One of these phenomena is so-called molecular mimicry , i.e. the imitation of proteins of the host organism by envelope proteins, which are no longer recognized by the immune system as foreign or can even imitate the functions of these proteins.
An example of this immunological camouflage is the similarity of parts of the coat protein of some virus species of the Coronaviridae family with the Fc fragment of the IgG antibody . The envelope protein E2 of the hepatitis C virus is structurally similar to part of the IgG antibody (ab fragment). In addition to such camouflage through structural imitation, the specific binding of host proteins to the virus envelope can also be used. This is the case in the case of the specific binding of albumin to the envelope of the hepatitis B virus.
In addition to the mere imitation of the host's own proteins for camouflage, the envelope proteins can also imitate the binding properties of the host proteins. In the case of the retroviruses of the genus Lentivirus , the similarity of the outer domain of the coat protein gp41 with interleukin-2 has been described; here, the binding to interleukin receptors is mimicked by immune cells that are the target cells of these viruses.
The ability of retroviruses in cell culture to induce budding without their own envelope protein is used in the genetic engineering of artificial virus particles in order to produce particles with modified surface properties. For example, foreign envelope proteins can be stored in the envelope of these so-called pseudotypes in order to be able to examine, for example, the binding of these to receptors or to use them as viral vectors in research. The formation of pseudotypes seems to be linked to the existence of the already mentioned lipid rafts .
The development of pseudotypes has also been described in natural infections. For example, two types of virus can store the different envelope proteins mixed in a newly emerging envelope when one cell is infected at the same time, or one virus can be packaged entirely with the envelope of the other virus. This phenomenon of pseudo-type formation is also called Phenotypic mixture ( "Phenotypic mixing" hereinafter).
Virus envelope and virus inactivation
The loss of the virus envelope or the removal of the lipid components from the envelope prevent the enveloped virus from infecting the host cell. This fact is used to inactivate enveloped viruses in order to prevent the virus from spreading. The most sensitive component of the virus envelope, the lipid membrane, can be destroyed by fat-dissolving alcohols such as ethanol or 2-propanol. If the virus envelope has a high lipid content, as is the case with orthomyxoviruses, mild detergents or soaps are sufficient to reduce the infectivity of the virus. A combination of mild solvents and detergents may be used in inactivating possible enveloped viruses such as HIV, HBV and HCV in blood products for transfusion .
Development of pandemics and "new viruses"
The high immunological flexibility of the envelope proteins allows some enveloped viruses to multiply in different host species. Infections can develop across species or intermediate hosts can be used as vectors. The viruses transmitted by arthropods ( e.g. mosquitoes and ticks ), the so-called arboviruses , are therefore predominantly enveloped viruses. The only non-enveloped genus Coltivirus , the members of which can be transmitted as arboviruses, have a second capsid to replace the flexibility of the virus envelope. Viruses are usually particularly pathogenic when they appear in a host population. Therefore, the enveloped viruses, which particularly favor the host transition from animals to humans, have a particularly high potential for new infections occurring in humans.
literature
Current literature
- Stephen C. Harrison: Principles of Virus Structure . In: David M. Knipe, Peter M. Howley et al. (eds.): Fields' Virology . 4th edition. Philadelphia 2001, ISBN 0-7817-1832-5 , pp. 53-85
- John AT Young: Virus Entry and Uncoating . In: Fields' Virology .
- SJ Flint, LW Enquist, VR Racaniello, and AM Skalka: Principles of Virology. Molecular Biology, Pathogenesis, and Control of Animal Viruses. 2nd Edition. ASM-Press, Washington DC 2004, ISBN 1-55581-259-7
- Joe Bentz (ed.): Viral Fusion Mechanisms . CRC-Press, Boca Raton 1993, ISBN 0-8493-5606-7
- Robert Brasseur (ed.): Molecular Description of Biological Membranes by Computer Aided Conformational Analysis . Vol. 1, CRC-Press, Boston 1990, ISBN 0-8493-6375-6
Historical literature
- Wolfhard Weidel: Virus - The story of borrowed life . Berlin / Göttingen / Heidelberg 1957
- Frank Fenner , BR McAuslan et al. (eds.): The Biology of Animal Viruses. Academic Press, New York / London, 1st edition 1968, 2nd edition 1974, ISBN 0-12-253040-3
- Alena Lengerová: Membrane Antigens . Fischer, Jena 1977
Web links
- Graph of the external and internal lipid components of HIV-1 (PNAS 2006)
- Cryo-EM analysis of the envelope of an alphavirus
Individual evidence
- ^ Karlheinz Lüdtke: On the history of early virus research . (PDF; 222 kB), MPI for the History of Science, 1999 (overview)
- ^ W. Reed: Recent researches concerning the etiology, propagation and prevention of yellow fever by the United States Army Commission . In: J. Trop. Med. , 1901, 5, pp. 143-158
- ↑ B. v. Borries, E. Ruska, H. Ruska: Bacteria and Virus in a microscopic image . (PDF; 925 kB) Klin. Wochenschrift , 1938, 17, pp. 921–925
- ↑ H. Ruska: Attempt to order the virus types . In: Arch. Ges. Virus research , 1943, 2, pp. 480-498
- ^ EW Goodpasture , AM Woodruff, GJ Buddingh: Vaccinal infection of the chorio-allantoic membrane of the chick embryo . In: Amer. J. Pathol. , 1932, 8, p. 271
- ↑ WIB Beverige, FM Burnet: The cultivation of viruses and rickettsiae in the chick embryo . In: Med. Res. Council Spec. Rept. Ser , 1946, p. 256
- ^ WB Dunham, WJ Macneal: Culture on the Chick Chorio-allantois as a Test of Inactivation of Vaccinia Virus . In: J. Bacteriology , 1942, 44 (4), pp. 413-424, PMID 16560579
- ↑ M. Uhler, S. Gard: Lipid content of standard and incomplete influenza A virus . In: Nature 1954, 173 (4413), pp. 1041-1042, PMID 13165714
- ↑ E. Gorter, F. Grendel: On bimolecular layers of lipoid on the chromocytes of the blood . In: J. Exp. Med. , 1925, 41, pp. 439-443, jem.org
- ↑ LH Frommhagen, NK Freeman, CA Knight: The lipid constituents of influenza virus, chick allantoic membrane and sedimentable allantoic protein . In: Virology , 1958, 5 (1), pp. 173-175, PMID 13519759
- ↑ LL Coriell, G. et al .: rake Electron microscopy of herpes simplex . In: J Bacteriol. , 1950, 59 (1), pp. 61-68, PMID 15400321
- ↑ S. Brenner, RW Horne: A negative staining method for high resolution electron microscopy of viruses . In: Biochim. Biophys. Acta , 1959, 34, pp. 103-110, PMID 13804200
- ^ D. Branton: Fracture faces of frozen membranes . In: PNAS , 1966, 55, pp. 1048-1056, PMID 5334198
- ^ SJ Singer, GL Nicholson: The fluid mosaic model of the structure of cell membranes . In: Science , 1972, 175, pp. 720-731, PMID 4333397
- ^ JW Corran, WC Lewis: Lecithin and Cholesterol in Relation to the Physical Nature of Cell Membranes . In: Biochem J . , 1924, 18 (6), pp. 1364-1370, PMID 16743417
- ^ RP Rand, V. Luzzati: X-ray diffraction study in water of lipids extracted from human erythrocytes: the position of cholesterol in the lipid lamellae . In: Biophys. J. , 1968, 8 (1), pp. 125-137, PMID 5641398
- ↑ JP Laliberte, LW McGinnes et al .: Integrity of membrane lipid rafts is necessary for the ordered assembly and release of infectious Newcastle disease virus particles . In: J. Virol. 2006, 80 (21), pp. 10652-10662, PMID 17041223
- ^ H. Imhoff, V. von Messling et al .: Canine distemper virus infection requires cholesterol in the viral envelope . In: J. Virology , 2007, 81 (8), pp. 4158-4165, PMID 17267508
- ↑ S. Hambleton et al .: Cholesterol dependence of varicella-zoster virion entry into target cells . In: J. Virology , 2007, 81 (14), pp. 7548-7558, PMID 17494071
- ↑ MK Jain, RC Wagner: Introduction to Biological Membranes . Wiley, New York 1980, ISBN 0-471-03471-1
- ↑ converted into molar percent from: RC Aloia et al .: Lipid composition and fluidity of the human immunodeficiency virus . In: PNAS , 1988, 85 (3), pp. 900-904, PMID 2829209
- ↑ calculated from Tables 1 and 4 in: O. Satoh et al .: Membrane structure of the hepatitis B virus surface antigen particle . In: J. Biochemistry Tokyo , 2000, 127 (4), pp. 543-550, PMID 10739944
- ↑ G. van Meer, K. Simons: Viruses budding from either the apical or the basolateral plasma membrane domain of MDCK cells have unique phospholipid compositions . In: EMBO J. , 1982, 1 (7), pp. 847-852, PMID 6329709
- ↑ N. Chazal, D. Gerlier: Virus Entry, Assembly, Budding, and Membrane Rafts . In: Microbiol. Mol. Biol. Rev. , 2003, 67 (2), pp. 226-237 (Review PMID 12794191 )
- ↑ BA, G. Meyers: The pestivirus glycoprotein Erns is anchored in plane in the membrane via an amphipathic helix . In: J. Biol. Chem. , 2007, E-pub, PMID 17848558
- ^ B. Rentier, EL Hooghe-Peters, M. Dubois-Dalcq: Electron microscopic study of measles virus infection: cell fusion and hemadsorption. J. Virol. , 1978, 28 (2), pp. 567-577, PMID 722861
- ↑ J. Torres, K. Parthasarathy et al .: Model of a putative pore: the pentameric alpha-helical bundle of SARS coronavirus E protein in lipid bilayers . In: Biophys. J. , 2006, 91 (3), pp. 938-947, PMID 16698774
- ↑ RH Cheng, RJ Kuhn et al .: Nucleocapsid and glycoprotein organization in an enveloped virus . In: Cell , 1995, 80 (4), pp. 621-630, PMID 7867069
- ^ RH Vogel, SW Provencher et al .: Envelope structure of Semliki Forest virus reconstructed from cryo-electron micrographs . In: Nature , 1986, 320 (6062), pp. 533-535, PMID 3960136
- ^ CH von Bonsdorff, R. Pettersson: Surface structure of Uukuniemi virus . In: J. Virology , 1975, 16 (5), pp. 1296-1307, PMID 52726
- ^ C. Risco et al .: Endoplasmic reticulum-Golgi intermediate compartment membranes and vimentin filaments participate in vaccinia virus assembly . In: J. Virol. , 2002, 76 (4), pp. 1839-1855, PMID 11799179
- ↑ for an overview of virus maturation and budding see: H. Garoff, R. Hewson, D.-JE Opstelten: Virus maturation by budding . In: Microbiol. Mol. Biol. Rev. , 1998, 62, pp. 1171-1190, PMID 9841669
- ↑ S. Tzlil, M. Deserno et al .: A statistical-thermodynamic model of viral budding . In: Biophys. J. , 2004, 86 (4), pp. 2037-2048, PMID 15041646
- ↑ DM Lerner, JM Deutsch, GF Oster: How does a virus bud? Biophys J. , 1993, 65 (1), pp. 73-79, PMID 8369463
- ↑ H. Garoff, K. Simons: Location of the spike glycoproteins in the Semliki Forest virus membrane . In: PNAS , 1974, 71 (10), pp. 3988-3992, PMID 4530279
- ↑ M. Delchambre et al .: The GAG precursor of simian immunodeficiency virus assembles into virus-like particles . In: EMBO J. , 1989, 8 (9), pp. 2653-2660, PMID 2684654
- ↑ TL Cadd, U. Skoging, P. Liljeström: Budding of enveloped viruses from the plasma membrane . In: Bioessays , 1997, 19 (11), pp. 993-1000, PMID 9394621
- ↑ D. Falke, R. Siegert, W. Vogell: Electron microscopic findings on the question of the double membrane formation of the herpes simplex virus . In: Arch Gesamt Virusforschung 1959, 9, pp. 484-496, PMID 13821428
- ↑ IL van Genderen, R. Brandimarti et al .: The phospholipid composition of extracellular herpes simplex virions Differs from did of host cell nuclei . In: Virology , 1994, 200 (2), pp. 831-836, PMID 8178468
- ↑ LM Stannard et al .: Electron microscopic study of the distribution of the Australia antigen in individual sera of 50 serologically positive blood donors and two patients with serum hepatitis . In: J. Clin. Pathol. , 1973, 26 (3), pp. 209-216, PMID 4700502
- ↑ CG Schüttler et al .: Variable ratio of hepatitis C virus RNA to viral core antigen in patient sera . In: J. Clin. Microbiol. , 2004, 42 (5), pp. 1977-1981, PMID 15131157
- Jump up ↑ F. Bonino, KH Heermann, M. Rizzetto, WH Gerlich: Hepatitis delta virus: protein composition of delta antigen and its hepatitis B virus-derived envelope . In: J. Virology , 1986, 58 (3), pp. 945-950, PMID 3701932
- ^ RA Crowther, NA Kiselev et al .: Three-dimensional structure of hepatitis B virus core particles determined by electron cryomicroscopy . In: Cell , 1994, 77 (6), pp. 943-950, PMID 8004680
- ↑ EL Oleszak et al .: Molecular mimicry between Fc receptor and S peplomer protein of mouse hepatitis virus, bovine corona virus, and transmissible gastroenteritis virus . In: Hybridoma , 1995, 14 (1), pp. 1-8, PMID 7768529
- ↑ YW Hu et al .: Immunoglobulin mimicry by Hepatitis C Virus envelope protein E2 . In: Virology , 2005, 332 (2), pp. 538-549, PMID 15680419
- ↑ JA Quiroga et al .: Inhibition of albumin binding to hepatitis B virions by monoclonal antibody to the preS2 domain of the viral envelope . In: Digestion , 1987, 38 (4), pp. 212-220, PMID 2452108
- ↑ PF Serres: Molecular mimicry between the trimeric ectodomain of the transmembrane protein of immunosuppressive lentiviruses (HIV-SIV-FIV) and interleukin 2 . In: CR Acad. Sci. III , 2000, 323 (11), pp. 1019-1029, PMID 11144025
- ↑ JA Briggs, T. Wilk, SD Fuller: Do lipid rafts mediate virus assembly and pseudotyping? J. General Virology , 2003, 84 (Pt 4), pp. 757-768 (Review PMID 12655075 )
- ↑ J. Dragunova et al .: Phenotypic mixing between vesicular stomatitis and Uukuniemi viruses . In: Acta Virol. , 1986, 30 (6), pp. 512-514, PMID 2881472
- ^ WR Moorer: Antiviral activity of alcohol for surface disinfection. Int. J. Dent. Hyg. , 2003) 1 (3), pp. 138–142 (Review PMID 16451513 )
- ↑ B. Horowitz et al .: Viral safety of solvent-detergent treated blood products . In: Dev. Biol. Stand. , 1993, 81, pp. 147-161 (Review PMID 8174797 )